J-PET (Jagiellonian-PET TOMOGRAPHY)
Jagiellonian PET is the first Positron Emission Tomography scanner build from plastic scintillators. J-PET, as a multi-photon PET, enables positronium imaging, quantum entanglement imaging and discreet symmetry studies. The most significant achievements made possible by the J-PET tomograph include:
- the first-ever positronium image in-vivo in the human brain [SciAdv2024] [download pdf],
- the first-ever positronium image ex-vivo [SciAdv2021] [download pdf],
- the first-ever multiphoton image [NatureComm2021] [download pdf],
- the best so far precision for CP studies in positronium decays [NatureComm2024] [download pdf],
- the best so far precision for CPT studies in positronium decays [NatureComm2021] [download pdf],
- the discovery that the degree of quantum entanglement of photons from positronium annihilation in material depends on the type of material [SciAdv2025] [download pdf].
The primary aim of the J-PET group is to develop a technology for cost-effective total-body PET based on plastic scintillators, which in contrast to inorganic ones, are relatively cheap and easy to shape [PETClin2020] [download pdf].
J-PET tomograph developement
The J-PET project began with a patent obtained by Prof. Paweł Moskal in 2009. Research on plastic scintillators for the construction of a PET scanner was initiated at that time. In 2014, the first prototype of the detector was constructed, a so-called small barrel, with 24 strips of plastic scintillator. Two years later, the first full-scale three-layer J-PET prototype was completed. In 2021, a modular, portable J-PET scanner was developed, which is successfully used in hospitals for patient scanning. A total-body J-PET scanner is currently being constructed based on the modular detector concept.
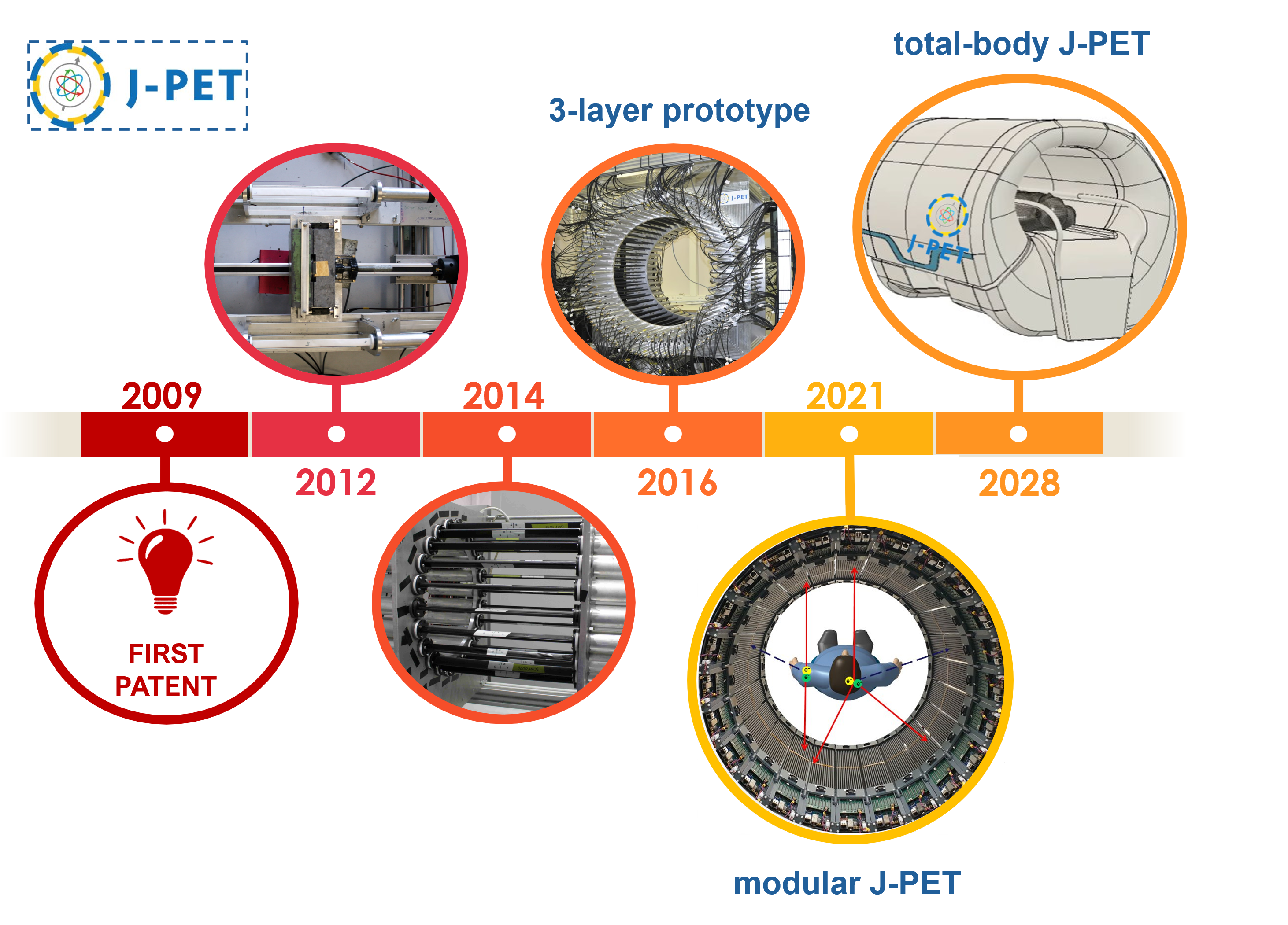
The J-PET tomograph development timescale.
Plastic scintillators are superior to the crystal ones in terms of their time resolution. The novelty of the J-PET detector lies also in the fact that the information about the place of gamma quanta interaction is extracted solely from the time, rather than the energy deposition measurement.
Full-scale J-PET prototype
The first full-scale J-PET prototype (3-layer) is shown in the photos below.
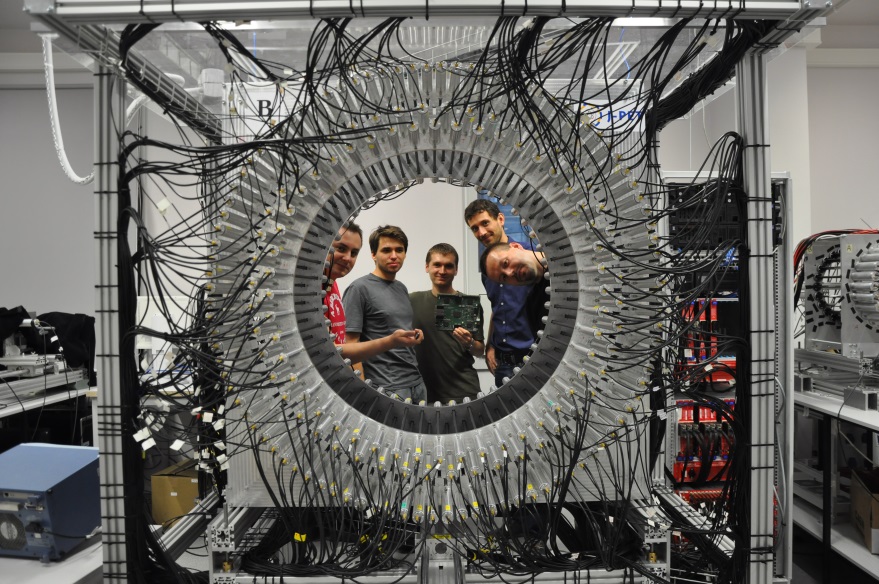
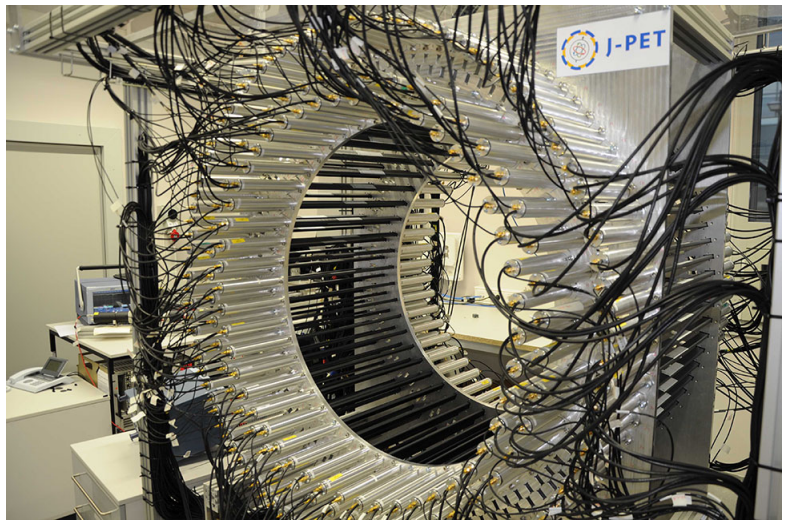
The first full-scale prototype of the J-PET detector. The J-PET detector is made of three cylindrical layers of EJ-230 plastic scintillator strips (black) with dimension of 7 × 19 × 500 mm3 and Hamamatsu R9800 vacuum tube photomultipliers (grey). The signals from photomultipliers are probed in the voltage domain at four thresholds with a timing accuracy of 30 ps and the data acquisition is working in the trigger-less mode. The prototype was built with the support from the Polish National Center for Development and Research through grant INNOTECH-K1/IN1/64/159174/NCBR/12.
In each of the 192 modules of the J-PET prototype detector there are two photomultipliers mounted at the both ends of a scintillator. Signals are sampled at four voltage levels at the leading and trailing edges with the newly developed, dedicated digital multi-threshold electronics [IEEETransMedImag2018][JINST2017]. The place of a gamma quantum interaction in the scintillator (Δl) is determined from the times measured at both ends of the same strip. For the determination of the position of the annihilation point along a line-of-response (Δx) a time-of-flight (TOF) method is used basing on the times registered in a pair of opposite strips [PhysMed2020]. Furthermore, timing of signals is used for suppression of scatterings via the the time-over-threshold (TOT) method [EJNMMI2020].
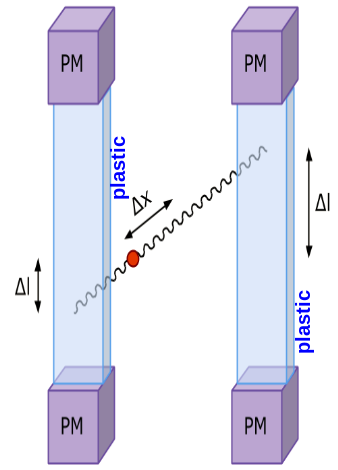
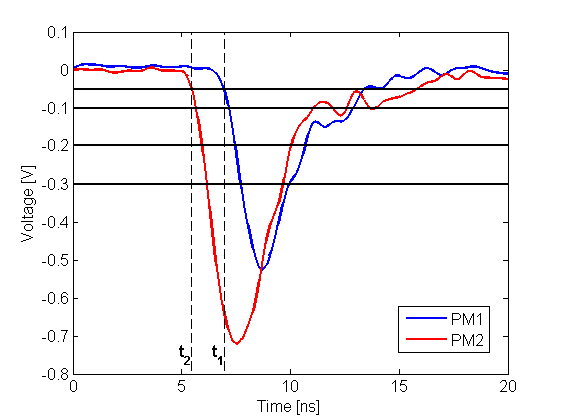
Left: The place of annihilation (marked as red spot) is estimated based on timing information gathered by two detection modules. First the interaction positions are determined based on time of arrival of light signal at both edges of plastic scintillator. This gives possibility to calculate delta L, distance from the center of a strip. Then one can connect two interaction positions constructing LOR (Line-Of-Response). Place of annihilation can be determined by comparison of interaction times between two modules. Right: Two exemplary signals from photomultipliers from each side of the same scintillator. Signal is measured at four thresholds, marked as horizontal black lines, at both edges. Such measurement gives opportunity to estimate place of interaction along the plastic strip as well as energy deposition of gamma quantum.
In order to compensate for the lower gamma quanta registration efficiency in the organic scintillators more detection layers can be used. This together with a large light attenuation length in the plastic scintillators allowed for construction of a tomograph with a large axial field-of-view, competitive in terms of spatial resolution and cheaper than commercially available scanners.
Furthermore, the J-PET constitues a high acceptance multi-purpose detecor optimized for the detection of photons from the positron-electron annihilation and can be used in the broad interdisciplinary investigations including, among others:
- positronium imaging; the first ex-vivo positronium image for Cardiac Myxoma and adipose tissue was obtained [SciAdv2021] [NatureRev2019],
- studies of discrete symmetries in the decays of positronium atoms [NatureComm2021] [NatureComm2024] ,
- quantum entanglement of high energy photons originating from the decay of ortho-positronium [SciRep2019] [arXive2407.08574],
- research in the field of life- and material-sciences [BioAlgMedSyst2021].
Modular J-PET tomograph
In the typical detectors with vacuum tube photomultipliers, only few first registered photons contribute to the leading edge of the electrical signal. Therefore, the time resolution may be improved by making a readout allowing to record timestamps from larger number of scintillation photons arriving at the scintillator edge. This can be achieved by the preparation of a a read-out system in the form of an array with several SiPM photomultipliers. In such a case, a set of all registered scintillation photons is divided into several subgroups and the time of the registration of the first photon in each subgroup is recorded.
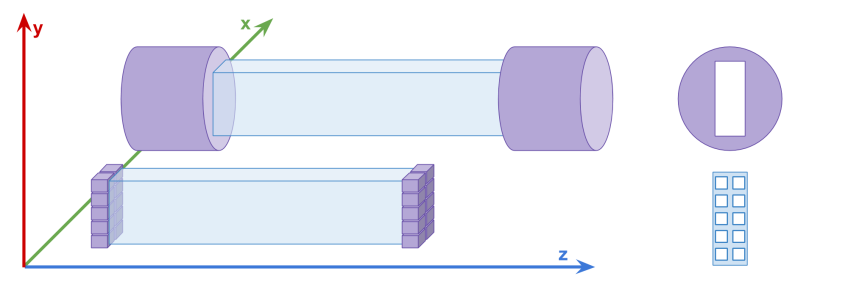
The upper diagram shows a single detection element of the first J-PET detector consisting of the scintillator strip read out on two sides by vacuum tube photomultipliers. The lower part shows a diagram of an exemplary multi-SiPM readout allowing for determination of timestamps of 20 detected photons (10 on each side).
Based on aforementioned concept, new detection modules were designed. A single module of the new detection layer of the J-PET tomograph is an independent detection unit which sends information about timing of the signals via optical links to the data acquisition boards. It consists of 13 BC-404 plastic scintillator strips. Each strip has dimensions of 6x24x50 mm3. Scintillators are wrapped in Vikuiti ESR and Pokalon 100B foils. Each side of scintillator strip is read out by S13361-6674 Hamamatsu SiPMs (Silicon PhotoMultipliers) in a form of a 1x4 matrix. SiPMs output is connected to the preamplifier and power supply boards. Supply board allows tuning of voltage for each SiPM separately with high precision, while preamp board first enhances the signals and then splits them passively into two. Analog signals are then fed into LVDS buffer of FPGA chip, with second voltage set to constant value. Such approach works as makes measurement of time at constant threshold possible for each detector channel separately. Conversion of analog to digital is performed at the end of the module to enable as high timing performance as possible. Digital signals are then handled by two stage digital readout system which can process part of the data stream on the fly and in the end store it on the server. Whole detector is build out of 24 detection modules mounted in a metal frame. Each module can be easily taken out for maintenance and weights about 2 kg in total.
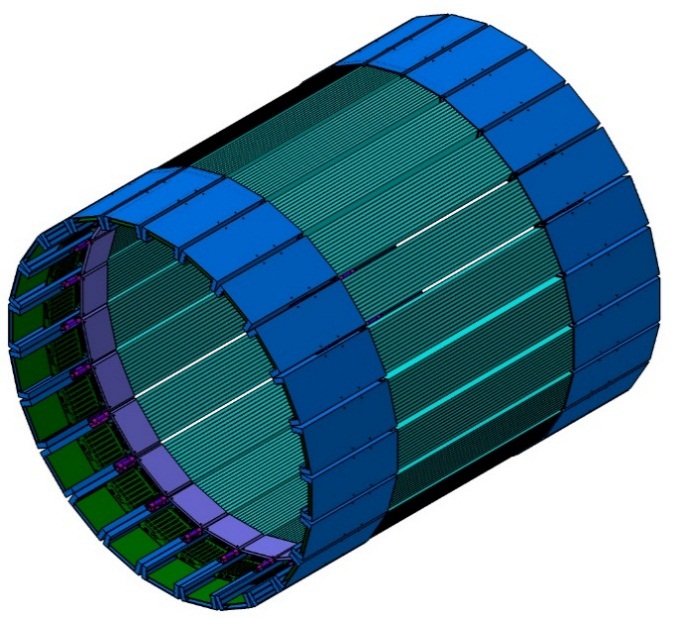
Due to the application of the matrix of the silicon photomultipliers, the new layer of the J-PET is characterized by even better timing resolution than the first prototype.
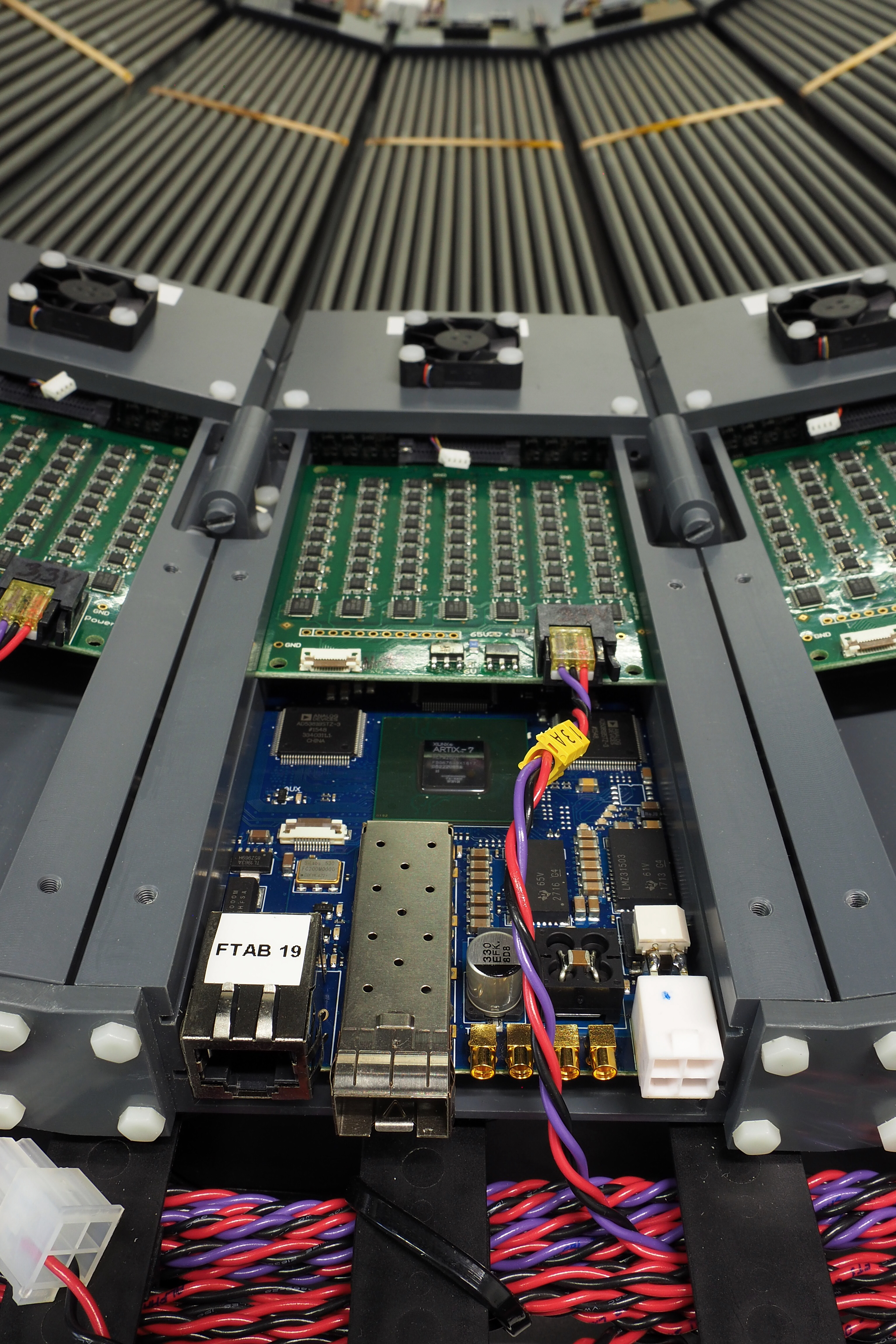
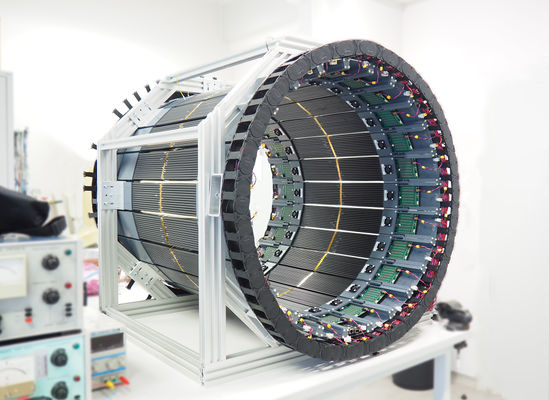
Left: Power supply board (green) providing voltage to each SiPM separately and TDC board (blue) converting analog signals to digital ones, containing the information of analog signal crossing at two selected constant thresholds. Right: Mobile J-PET prototype after mechanical assembly. Detector is build out of 24 detection modules. Each module can be easily taken out for maintenance and weights about 2 kg in total and consists of 13 scintillators read out at both sides by arrays of 1x4 SiPMs. Analog signals are digitized as close as possible to the SiPMs using TDC boards developed by the J-PET group.
The modular J-PET tomograph is multidisciplinary detection system used for medical imaging as well as for fundamental studies (e.x. discreet symmetries tests, photon entanglement studies). Beyond the standard PET imaging, the J-PET scanner allows for positronium lifetime imaging in the patient body. The first ever in-vivo image of positronium in the human brain was obtained recently with J-PET [SciAdv2024] and shows the great potential of this new diagnostic method in the future.
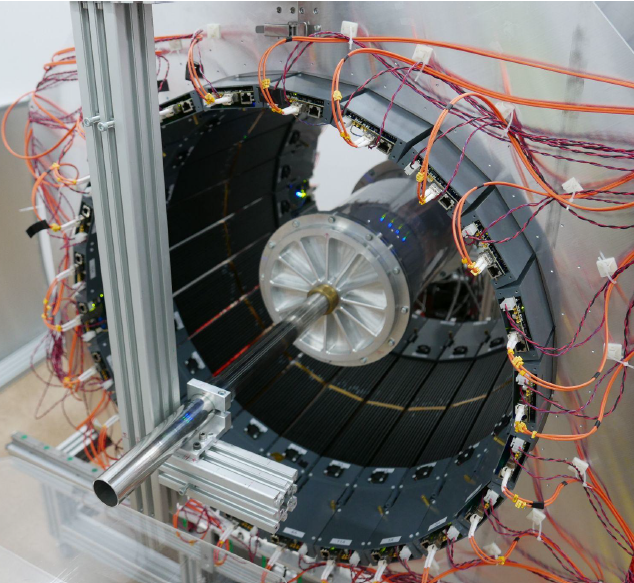
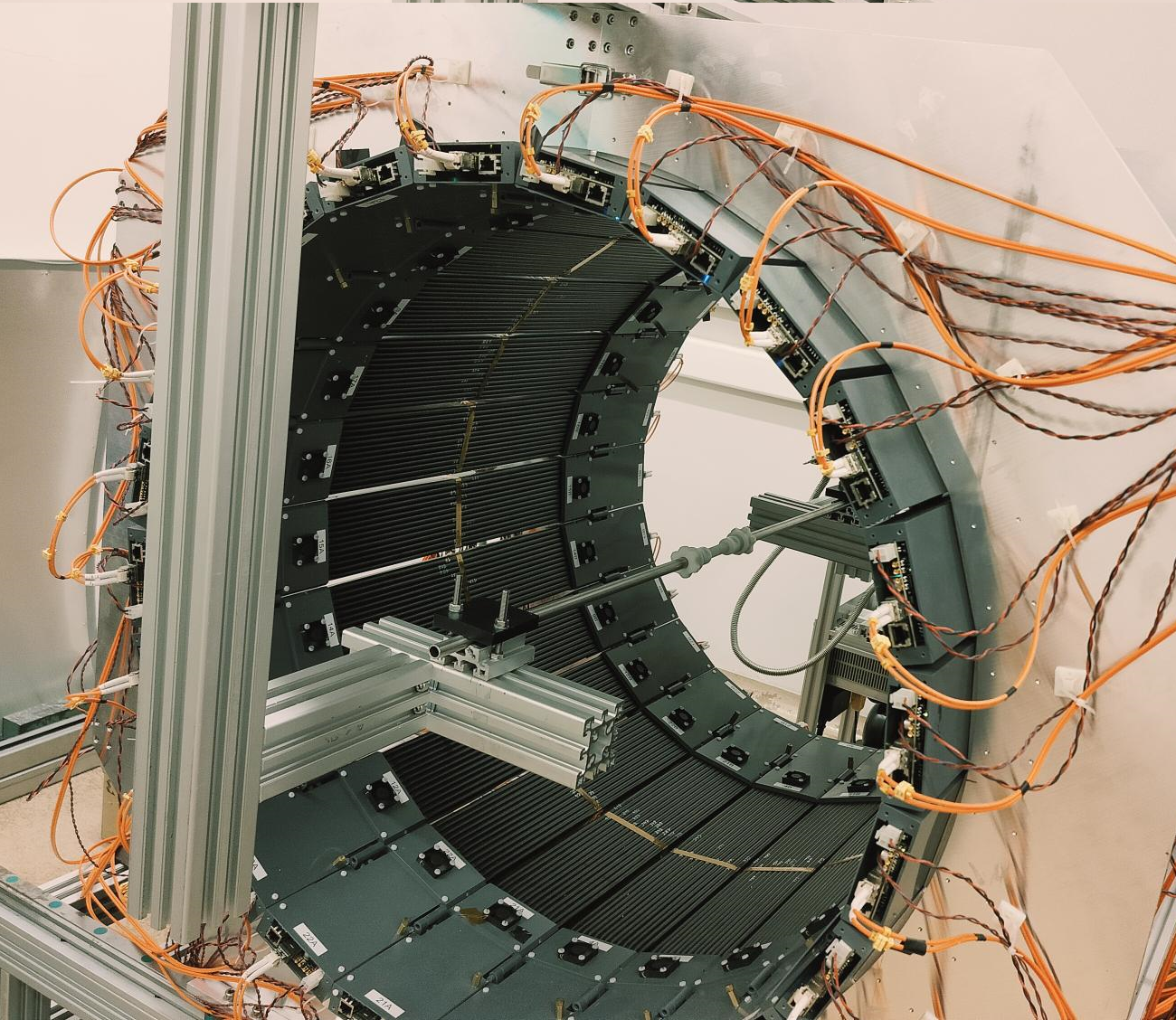
The modular J-PET tomograph with an installed spherical annihilation chamber (left) and a small cylindrical annihilation chamber (right) used for the fundamental research.
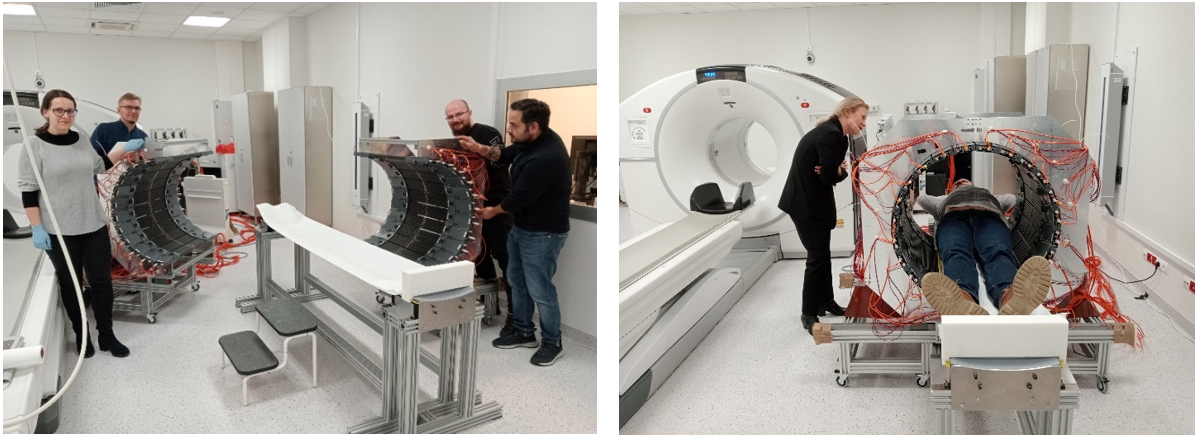
Measurements in the University Hospital in Kraków in Spring 2024 (Bioethics Committee consent no 1072.6120.92.2023). (left) J-PET members prepare the tomograph for patient scan. (right) Patient measurement with J-PET modular tomograph.
J-PET group history
The Jagiellonian PET collaboration lead by P. Moskal is an interdisciplinary and international group including physicists, chemists, electronic engineers, computer scientists, quantum information physicists as well as bio and medical physicists from the Jagiellonian University, Institute of Nuclear Physics - Cyclotron Centre Bronowice, AGH University of Science and Technology, Cracow University of Technology, Institute of Nuclear Chemistry and Technology, Heavy Ion Laboratory, University of Warsaw, Maria Curie-Skłodowska University, John Paul II Hospital in Krakow, University Hospital in Krakow, National Laboratory in Frascati and from the companies Nowoczesna Elektronika and Brain Waves Electronics.
Positronium imaging
The J-PET detector is the first detector capable of positronium imaging. The positronium imaging is a concept, proposed by the founder of the J-PET group P. Moskal, that combines two kind of information that can be extracted from a positronium decay – position of the decay and the positronium lifetime. In particular, the lifetime of relatively long-lived o-Ps is an extremely precise structural indicator that has been extensively studied in the context of material and surface research. Changes in the mean o-Ps lifetime enables the characterization of the porosity of the material in the range of 1-100 nm.
The first positronium image was created by studying three-photon coincidences, where two photons were coming from the positronium annihilation and the third photon was coming from the deexcitation of the positron emitter nucleus (22Na → 22Ne* + e+ + νe; 22Ne* → 22Ne + γdeex). Annihilation photons from the two-photon decay of the positronium allows for the determination of the annihilation position, where the registration of the deexcitation photon allows for measurement of single lifetimes of the positronium. Simultaneous measurement of the annihilation position and positronium lifetime allowed to form image consisting of the o-Ps lifetime estimated in each voxel – positronium image. The first-ever positronium image ex-vivo for phantoms constructed from human cardiac myxoma and adipose mediastinal tissues was reconstructed based on measurements with the 3-layer J-PET prototype [SciAdv2021].
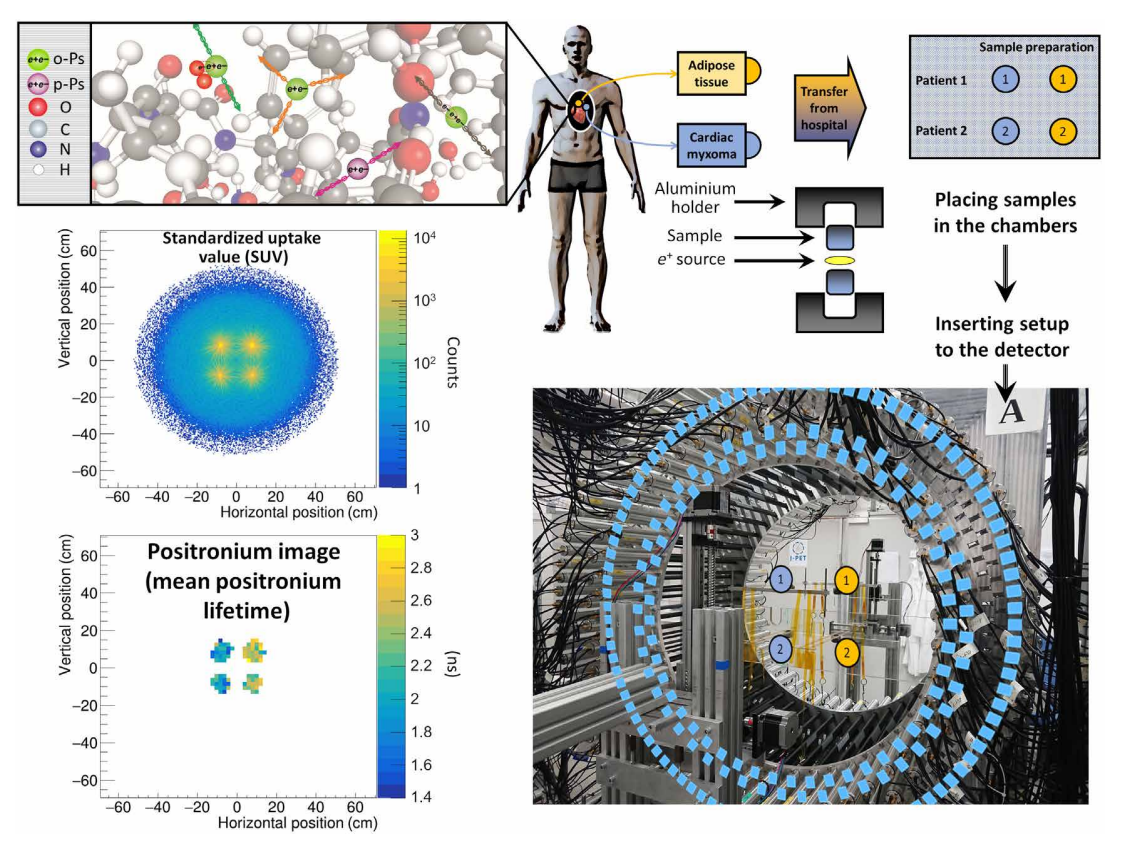
The measurement scheme and the determined SUV and positronium images. The top left panel illustrates a part of a hemoglobin molecule with superimposed schemes, indicating decays of o-Ps (green circles) and parapositronium (p-Ps) (violet circle). o-Ps may undergo self-annihilation (pink arrows), pickoff process (gray arrows), or conversion to parapositronium, e.g., by interacting with oxygen molecule (green arrows). The top right panel shows the first part of the experimental workflow. Four samples from two patients were collected and divided into two classes: cardiac myxoma and adipose tissue. Each sample was inserted into a holder with the radioactive 22Na source, which was inserted into the J-PET detector. The blue and yellow circles show the locations of the samples during the measurement. Methods to reconstruct the image analogs to that of the SUV image (annihilation rate distribution) and positronium lifetime image are described in Methods. Reconstructed mean o-Ps lifetime in cardiac myxoma (1.9 ns) differs from the mean o-Ps lifetime in adipose tissue (2.6 ns). More comprehensive studies confirming the differences in the o-Ps mean lifetime in the healthy adipose tissue and cardiac myxoma tumor are described in the article to be submitted elsewhere.
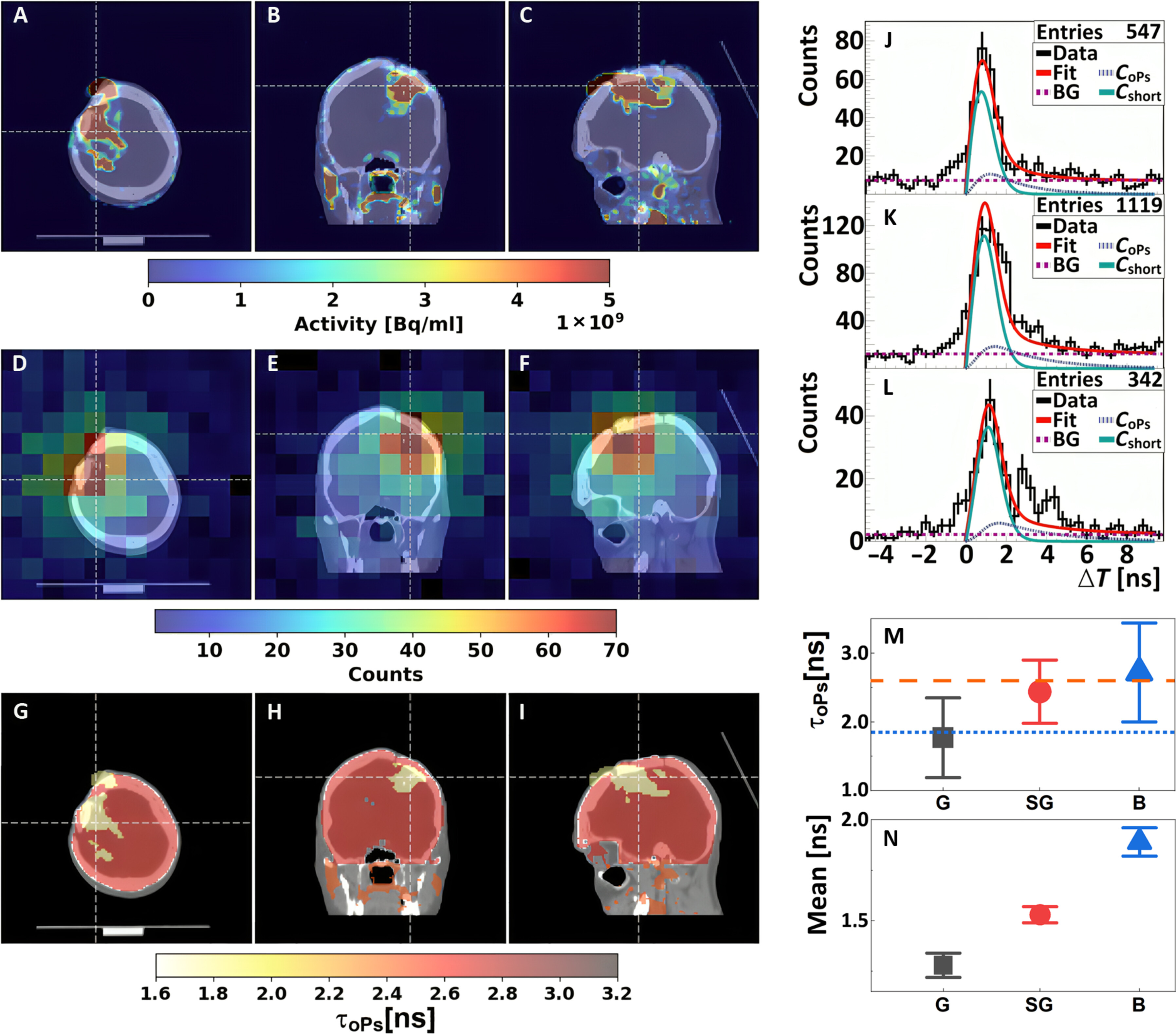
Positronium images of the head of a patient with recurrent secondary glioblastoma in the right frontoparietal lobe. (A to C) Standard PET/CT images obtained with Biograph 64 TruePoint. An accumulation of [68Ga]Ga-DOTA-SP in the capsule and cavity of the tumor in transverse (A), coronal (B), and sagittal (C) planes is presented. (D to F) Images of the density distribution of positron annihilation accompanied by the emission of the prompt gamma are shown in transverse (D), coronal (E), and sagittal (F) planes. These images were acquired with the J-PET tomograph. The white thin dashed lines in each (A) to (F) image indicate a cross section with the other two planes presented. (G to I) Positronium images shown in transverse (G), coronal (H), and sagittal (I) planes. (J to L) Distributions of positron annihilation lifetime (ΔT) determined in the glioblastoma tumor (J), salivary glands (K), and healthy brain tissues (L). Black histograms denote experimental data. The superimposed curves indicate the result of the fit of the function describing contributions from oPs (CoPs), from pPs and direct annihilations (Cshort), and from the background due to accidental coincidences (BG). The red curve denotes the sum of all contributions (Fit). (M and N) Mean oPs lifetime [τoPs; (M)] and mean value of the positron lifetime in the range between 0 and 5 ns [Mean; (N)] determined for the glioblastoma tumor (G; black squares), salivary glands (SG; red circles), and healthy brain tissues (B; blue triangles). The blue dotted line and orange dashed line indicate the mean oPs lifetime in cardiac myxoma tumor (1.92 ns) and adipose tissue (2.72 ns).
The mean-lifetime positronium image is a complementary image to the standard PET image, which can extend not only the diagnostic capabilities of the PET technique, but also the applicability of PET scanners for example in material research. Additional structural information derived from the measurement of mean o-Ps lifetime may help in the detection of neoplastic lesions not detected with standard PET scans as well as in determining their degree of malignancy, and therefore constitute the so-called “virtual biopsy”.
More information can be found in:
Multiphoton imaging
The ability of the J-PET detector to reconstruct the decays of ortho-positronium (o-Ps) atoms into three photons was proven. The method is based on trilateration (GPS-like method) and allows for a simultaneous reconstruction of both location and time of the decay [NIMP2016]. Altough gamma quanta interact in the plastic scintillators predominantly via the Compton effect, making a direct measurement of their energy impossible, it was shown that the J-PET scanner will enable studies of the o-Ps→3γ decays with angular and energy resolution equal to σ(θ) ≈ 0.4o and σ(E) ≈ 4.1 keV, respectively.
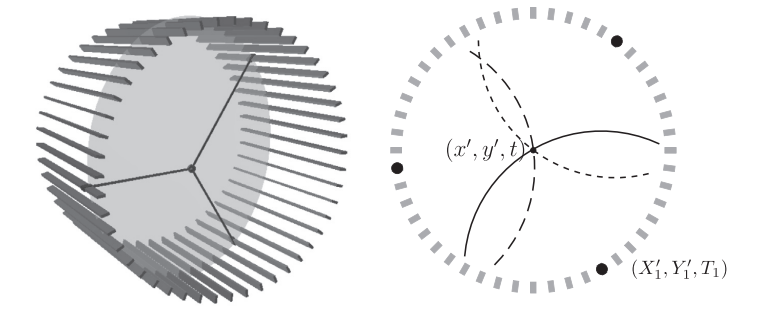
Left: A diagram of the J-PET detector with gamma quantum hit positions marked in black and the o-Ps decay plane indicated in gray. Right: Diagram of the decay reconstruction reduced to a two-dimensional problem in the decay plane. Each of hit coordinates constitutes the center of a circle describing possible photon origin point, where radius of the circle depends on the recording time and the unknown o-Ps decay time.
The first imaging of o-Ps→3γ annihilation points using trilateration method was conducted for measurement with the cylindrical annihilation chamber devoted to CPT symmetry studies [NatureComm2021]. The tomographic image of the cylindrical chamber has been reproduced.
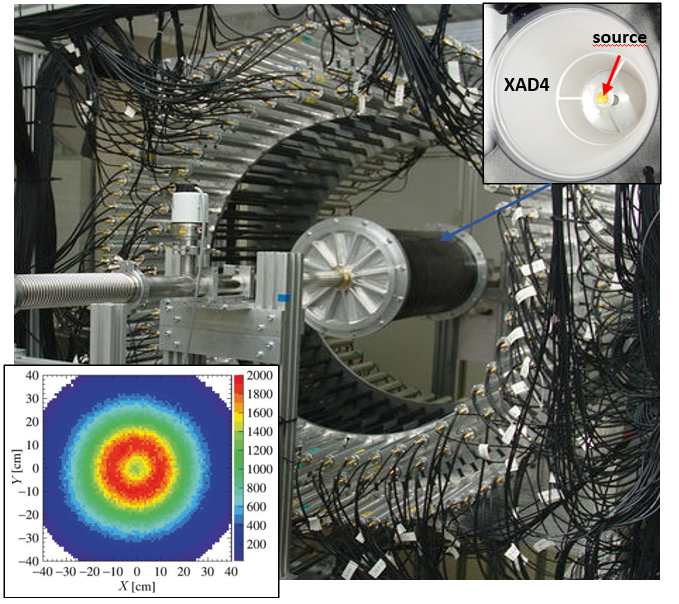
Photo of the J-PET prototype tomograph with the cylindrical positronium production chamber with a sodium 22Na radioactive β+ source placed at the center (see right upper pad). Reconstructed spatial locations of the identified o-Ps → 3γ events obtained using three-photon annihilations, allowing us to reproduce a tomographic image of the vacuum chamber (see left bottom pad). The maximum density distribution is in a ring with a radius of 12 cm, equal to the radius of the positronium production chamber.
More information can be found in:
- Testing CPT symmetry in ortho-positronium decays with positronium annihilation tomography
- Trilateration-based reconstruction of ortho-positronium decays into three photons with the J-PET detector
- A feasibility study of ortho-positronium decays measurement with the J-PET scanner based on plastic scintillators
- Feasibility study of the positronium imaging with the J-PET tomograph
- Positronium in medicine and biology
- The J-PET detector-a tool for precision studies of ortho-positronium decays
Quantum entanglement studies
Entanglement is a fundamental phenomenon in quantum mechanics, where two or more particles become interconnected, such that the state of one particle influences the state of the other particles instantly, regardless of the distance between them. Albert Einstein famously described this phenomenon as "spooky action at a distance," highlighting its counterintuitive nature and its challenge to classical physical intuitions. Our research explores quantum entanglement by focusing on the decay of positronium, a short-lived bound state consisting of an electron and its antiparticle, the positron. Upon annihilation, positronium emits pairs of photons that exhibit strong entanglement, governed by the conservation of angular momentum and parity. Specifically, the spin-0 (singlet) state of positronium mandates that the emitted photons possess opposite angular momenta, with their polarization vectors remain orthogonal.
The photons produced in positronium annihilation fall into the keV range, measuring the polarization using techniques developed for optical photons will not work. However, the Klein-Nishina (KN) formula provides a potential method for determining photon polarization by correlating the scattering angle (θ) with the azimuthal angle (η), where η represents the angle between the scattering plane and plane of polarization. The scattering plane is defined by the momentum vectors of incident (k) and scattered ((k')) photon, while the polarization plane is represented by k and its linear polarization vector (ε). According to KN, the differential cross-section reaches its maximum value at η = 90o. Therefore, the polarization direction is determined by the cross product of k and (k'), which defines the normal to the scattering plane (Fig. 1a). The J-PET detector, which utilizes plastic scintillators, is ideal for measuring the photons polarization specifically optimized for the precise detection of photons undergoing Compton scattering, achieving an angular resolution of approximately 2o (see Fig. 1b).

Compton scattering as polarization analyser: (a) An incident photon with momentum k is scattered by an electron, resulting in a change of its momentum in (k'). The angle of Compton scattering is denoted by θ. The initial (k) and scattered ((k')) momentum of the photon define a plane called the scattering plane. In addition, the momentum of the incident photon (k) and its linear polarization vector (ε) form another plane, the so-called polarization plane. The angle between those two planes is referred to as η. (b) shows a single photon interaction in the J-PET geometry, which allows detection of photon both before and after scattering. This enables precise measurement of the scattering angle (θ).
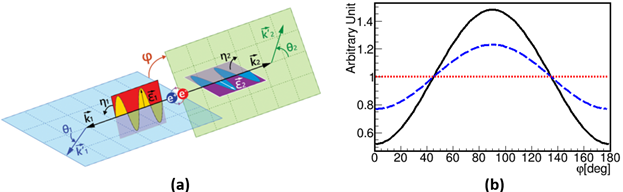
Polarization correlation of annihilation photons: The left panel (a) illustrates the Compton scattering of annihilation photons originating from para-Positronium (p-Ps) decay, highlighting the scattering angles (θ1 and θ2) and the associated scattering and polarization planes. The angles η1 and η2 show the angles between the scattering and polarization planes, while 𝜑 denotes the angle between the scattering planes, reflecting the relative orientation of the polarization planes of the photons. Panel (b) shows the angular distribution of φ, the angle between the scattering planes of photons, where both photons are scattered with θ_1=θ_2 ~ 82o. The solid black curve corresponds to entangled photon pairs, the dashed- blue curve represents independent Compton interactions of photons, and the red dotted line indicates uncorrelated photon polarizations, as in the case of photons from separate decays.
More information can be found in:
- Nonmaximal entanglement of photons from positron-electron annihilation demonstrated using a novel plastic PET scanner
- Feasibility studies of the polarization of photons beyond the optical wavelength regime with the J-PET detector
- Colloquium: Positronium physics and biomedical applications
- Positron Emission Tomography Could Be Aided by Entanglement
Hadron therapy range monitoring
The main advantage of the proton therapy over the conventional radiotherapy (which uses X-ray or electrons) is the possibility to precisely deposit the radiation dose only in the tumor region. This is a consequence of the characteristic energy loss distribution of ionizing radiation when the proton beam moves through matter. The maximum of energy deposition is localized immediately before the maximum movement range (the Bragg peak).
Altought the range of the proton beam in a homogenous medium of know properties can be precisely calculated, the expected range in the patient's body is determined only with limited precision. Therefore, in order to cover the entire volume of tumor a margin of healthy tissues surrounding it are also exposed to radiation.
The availability of beam range monitoring would allow for more precise therapy planning and for a more complete usage of proton therapy benefits.
The result of proton beam interactions with the atoms in the patient's tissues is the emission of the secondary particles, mainly: neutrons, photons (promt-gamma) and charged particles. Additionally, due to the nuclear interaction isotopes which decay via the β+ decay are created. Emited positrons annihilate with the electrons from the patients body and two back to back gamma quanta with a minimum energy of 511 keV are emitted. These gamma quanta can escape from the patient's body and be registered [ZMedPhys2022] [PhysMed2022]. The aim of the project developed in colaboration with the Institute of Nuclear Physics of the Polish Academy of Sciences is to test the usefulness of the J-PET technology for the monitoring of the proton beam range.
In the middle of the October 2021 the experiment aimed at measuring the range of irradiation using proton beam in different phantoms was conducted. In the facility where the experiment was conducted mainly tumors located in inoperable positions are treated, especially those located very close to vital organs, e.g. brain. Thus knowing exact depth of proton interaction and possibility to modify it in a fast way is pivotal.
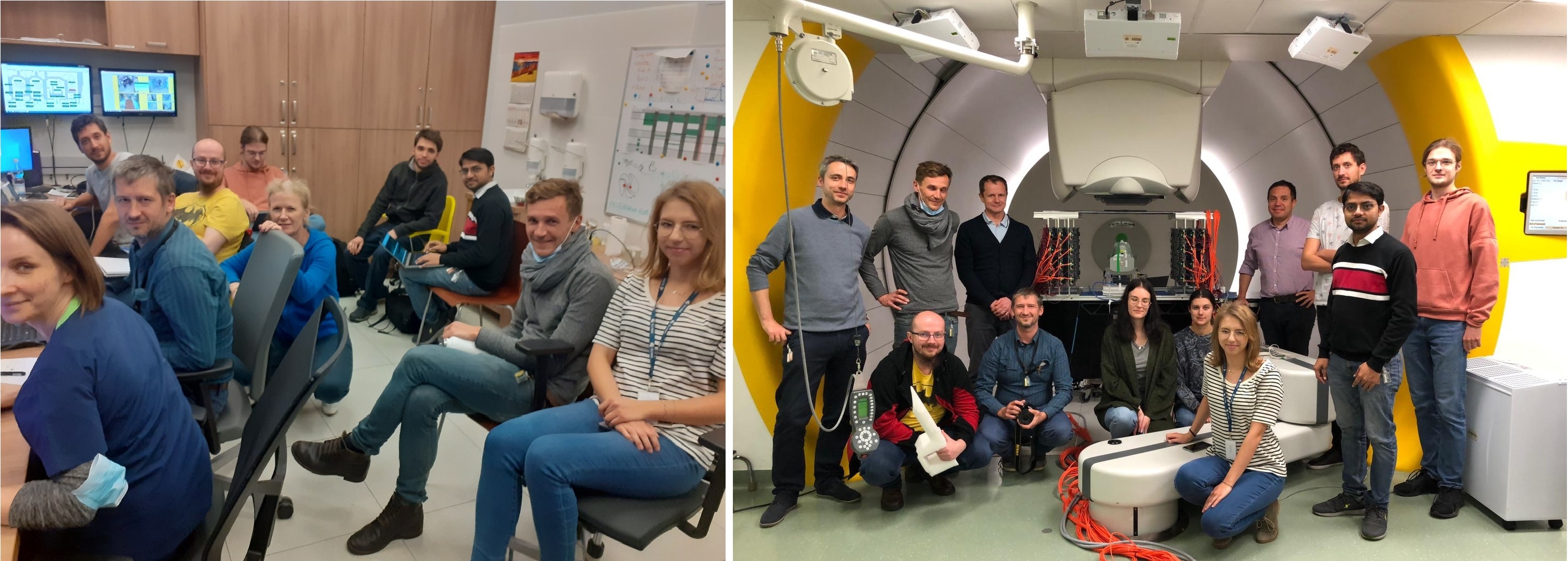
J-PET members in the Institute of Nuclear Physics of the Polish Academy of Sciences.
The modular J-PET detector was arranged in a 3 layers dual-head setup with 24 modules as shown in Fig. 1A, 1B. The uniform, water equivalent gel phantom was irradiated with four, homogeneous 16 Gy SOBP fields of 100 mm, 104 mm, 110 mm, and 119 mm range corresponding to 117.77 MeV, 120.53 MeV, 124.23 MeV, 129.85 MeV distal proton beam energy, respectively (see Fig.1C). The continuous data acquisition during the beam on and between the subsequent field irradiations was performed. Processed data were formed in a coincidences list. PET images were reconstructed with the CASToR software (MLEM, 10 iterations).
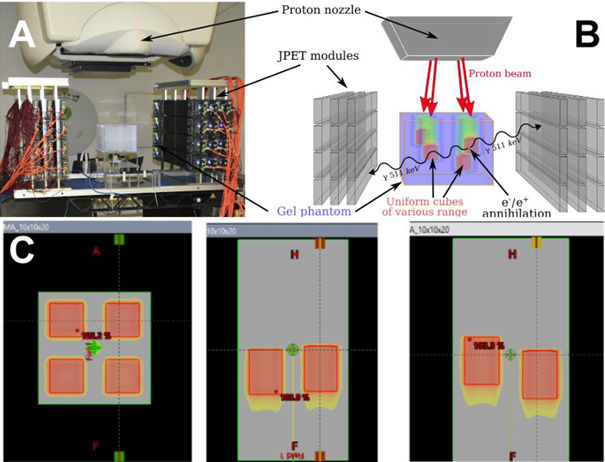
Fig1: Panel A: The experimental setup with the J-PET detector, proton nozzle, and gel phantom. Each layer in the head has 4 modules. Each module consists of 13 50-cm-long scintillator strips. The annihilation gamma deposits its energy via Compton scattering producing the scintillation which is propagated to the ends of the strips, converted to the electronic signal by SiPM, and processed with FPGA. Panel B: scheme of the experiments indicating the position of the irradiation fields. Panel C: Irradiation plans of SOBP fields of different ranges.
In above figure the exemplary reconstructed images of the activity gathered during the PET acquisition are presented for the SOBP field with the range of 100 mm. The reconstructed activity profiles along the beam direction for each of the fields are depicted in Fig. 2B. The differences in the proton beam ranges are clearly visible between Fields 1,2 and 3, demonstrating the feasibility of the J-PET system to detect beam range variations. The acquisition for field 4 was interrupted as ¼ of the scanner was not working properly due to the DAQ glitch and could be potentially biased. The PET image quality could be improved by applying normalization, random, and scatter corrections. A characteristic elongated activity distribution visible along the x-direction is due to non-cylindrical detector geometry; LORs were collected only by two opposing detector heads. including attenuation and sensitivity corrections. Reconstructed activity profiles for each field were compared to assess the feasibility of the modular J-PET for range monitoring.
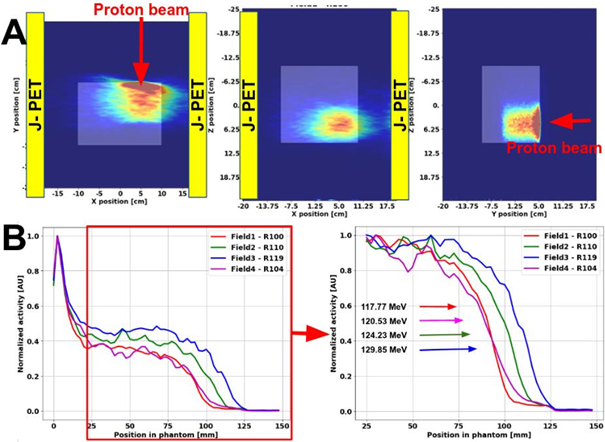
Fig2: Panel A: The reconstructed PET activity distribution for the SOBP with the range of 100 mm. Positioning of the J-PET detector heads (yellow rectangular) and proton beam direction is indicated. Panel B: The normalized reconstructed activity profiles for SOBP fields of different ranges. Substantial differences in activity range between fields are noticeable.
The results show for the first time that the J-PET detector is feasible to acquire β+ signal produced during the proton beam irradiation and detect a few millimeter range variations of homogeneous dose fields in a uniform phantom. Further investigations include improvements in data processing, image reconstruction, and a more detailed assessment of the J-PET system precision for proton beam range detection in the clinical setting.
More information can be found in:
- Feasibility of the J-PET to monitor the range of therapeutic proton beams
- Estimating influence of positron range in proton-therapy-beam monitoring with PET
- ProTheRaMon - a GATE simulation framework for proton therapy range monitoring using PET imaging,
- J-PET application as a Compton camera for proton beam range verification: A preliminary study
- Detection of range shifts in proton beam therapy using the J-PET scanner: a patient simulation study
- Experience and new prospects of PET imaging for ion beam therapy monitoring
Positronium as a cancer biomarker
We are aiming for examining a possible biomedical application of positronium for the characterization of normal and cancer cells and tissues, hence if it can serve as a cancer biomarker. Studies were conducted on two models: benign cardiac myxoma specimens and malignant melanoma cultured cell lines. In both cases, positronium properties were compared to an appropriate normal tissue and cell line. Studies are performed for both fixed and living cells and tissues to investigate the influence of water and cell viability on the PALS signal. Obtained results show significant differences in positronium lifetime and its production intensity between cancer and normal cells and tissues in all studied cases, regardless of hydration and fixation of specimens.
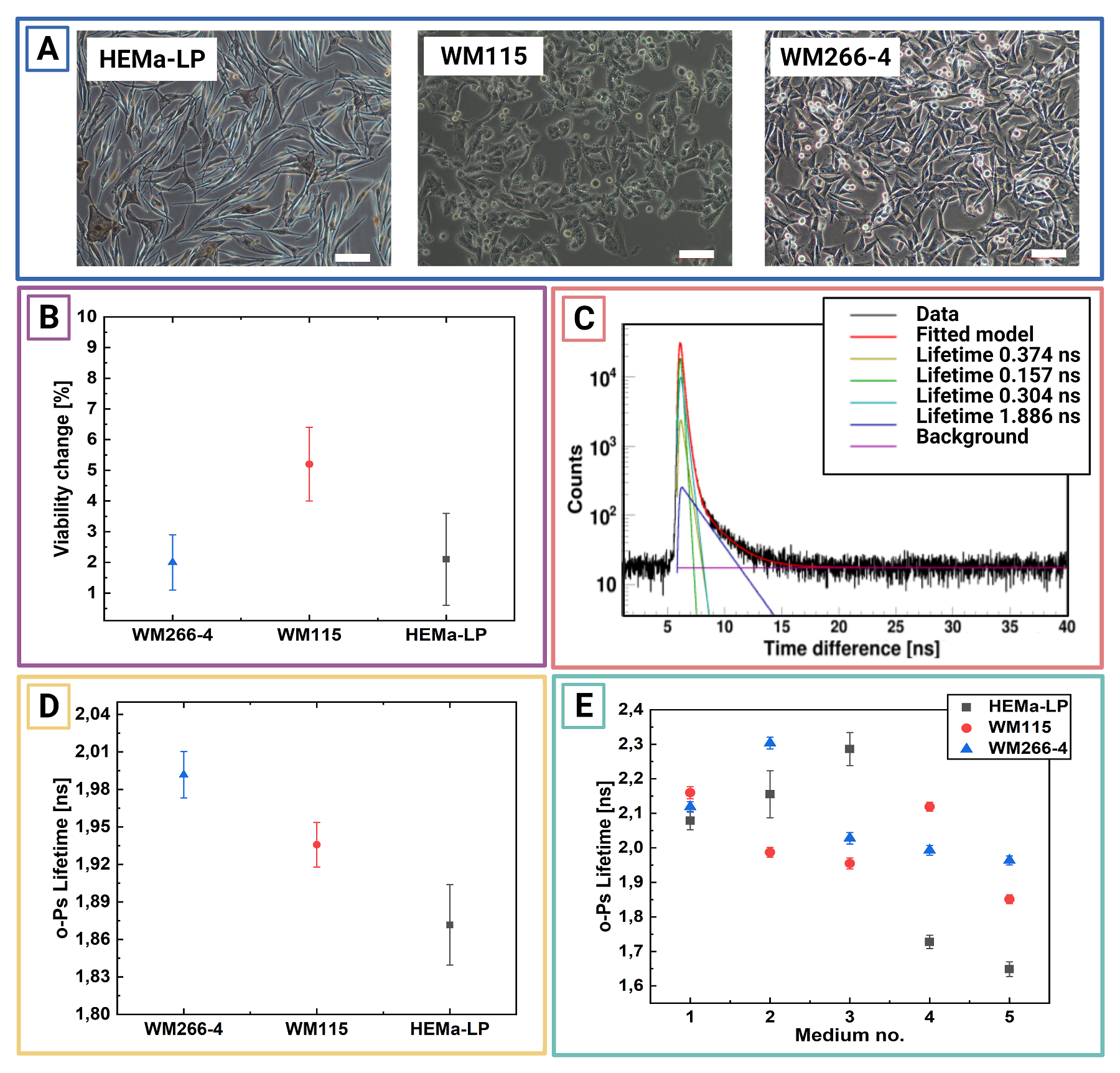
Micrograph showing the culture of HEMa-LP, WM115, and WM266-4 cell lines. Scale bar has100µm. B:Exemplary PAL spectra for freeze-dried inmedium no. 5 WM115cell culture.Superimposed linesindicate distributions of particular components resulting from thefitted model. Green - para-Positronium, yellow - annihilation in the source material, turquoise - free positron annihilation, blue - ortho-Positronium, purple – background. The spectra do not start at zero because of the time offset between the detectors. C: Mean ortho-positronium lifetime for freeze-dried in different media melanocytes (HEMa-LP) and melanomas (WM115, WM266-4) cell culture.D: Viability change before and after PALS measurement for living cell culture studies. Each point is calculatedas an average anduncertainty as a standard deviation from3 consecutivemeasurements. E: Mean ortho-positronium lifetime for vital melanocytes (HEMa-LP) and melanoma (WM115,WM266-4) cell culture. Each point is calculated as an average and uncertainty as a standarddeviation from 3 consecutive measurements.
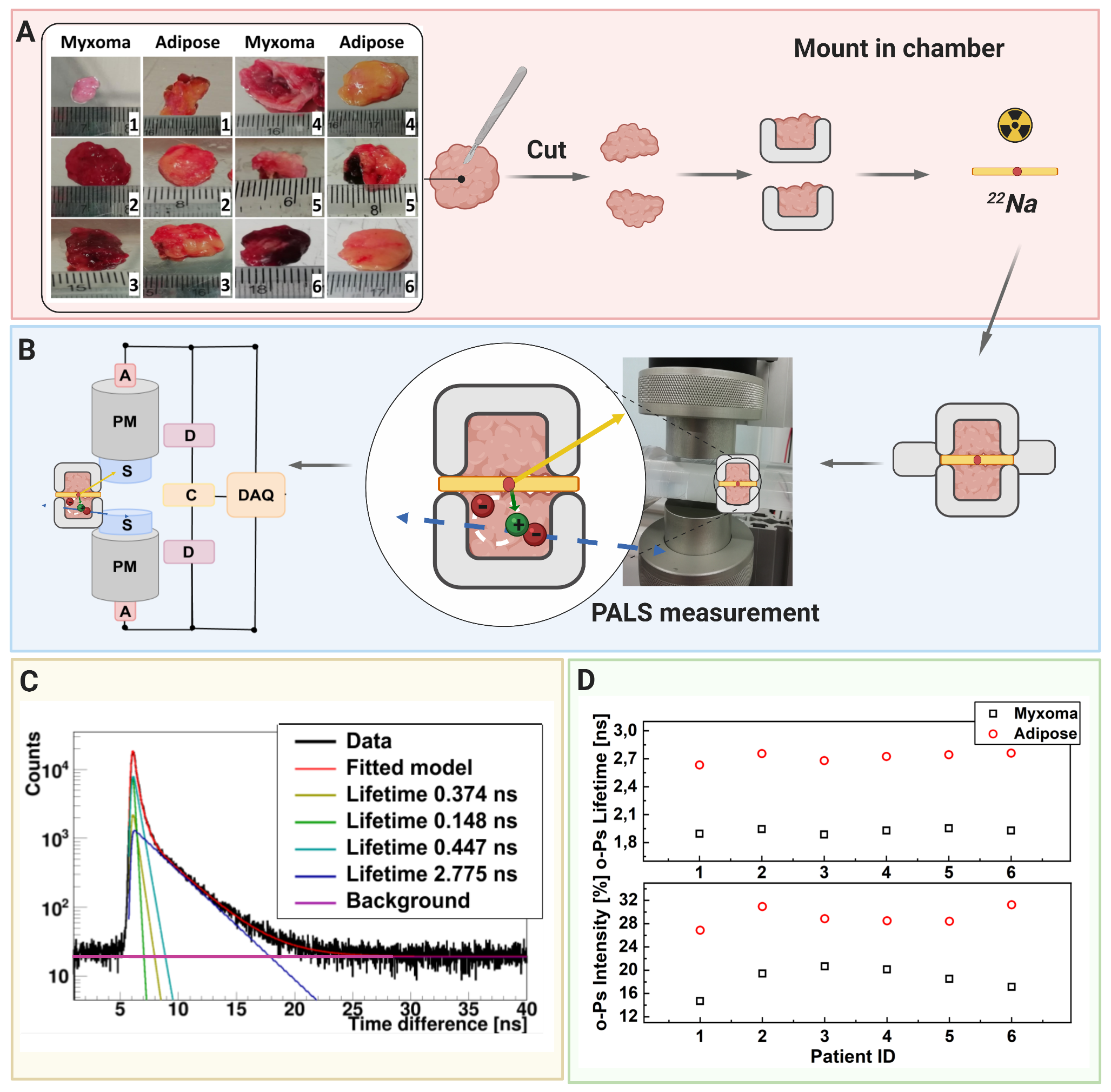
A scheme showing positronium lifetime measurements in cardiac myxoma and adipose tissues. (A) Photographs of non-fixed cardiac myxoma (CM) and adipose tissue samples. The numbers indicate patient ID. Each sectioned tissue has been cut in halves with a 22Na radionuclide placed between and inserted into the aluminum measurement chamber. (B) The left part of the panel depicts the scheme of the detection system: scintillators (S), photomultipliers (PM), attenuators (A), discriminators (D), coincidence units (C), digitizer, and a data acquisition system (DAQ). The photograph displays a part of the system together with the plastic rod localized between the scintillators. The superimposed scheme indicates an aluminum chamber inserted inside the rod. 22Na (red dot) emits (green arrow) positron (+), which annihilates (predominantly into two photons indicated in blue) with electrons (-) in the tissue. Following the positron emission, 22 Na changes into an excited nucleus of 22Ne, which deexcites almost instantly by the emission of the de-excitation photon (indicated in yellow). The PALS detection system, enables the measurement of the positronium lifetime by registering the time of emission of the deexcitation photon (corresponding to the time of positronium formation) and the time of creating the annihilation photons (corresponding to the time of the positronium decay). (C) For each sample, 1 x 106 coincidences between annihilation and deexcitation gamma quanta have been registered, resulting in the lifetime spectrum (example for the adipose tissue - patient ID 2). The analysis enables the extraction of the mean lifetime and intensities of para-Positronium (green line) and ortho-Positronium (blue line) atoms trapped in the intra-molecular voids . The dark yellow, turquoise, and purple lines denote direct annihilation in the source material, direct annihilation in the sample, and the background because of the accidental coincidences, respectively. The spectra are shifted by the delay coming from detection system configuration (~5ns). (D) Results of the mean ortho-Positronium (o-Ps) lifetime (upper) and intensity (lower panel) for CM (black squares) and mediastinal adipose (red circle) tissues
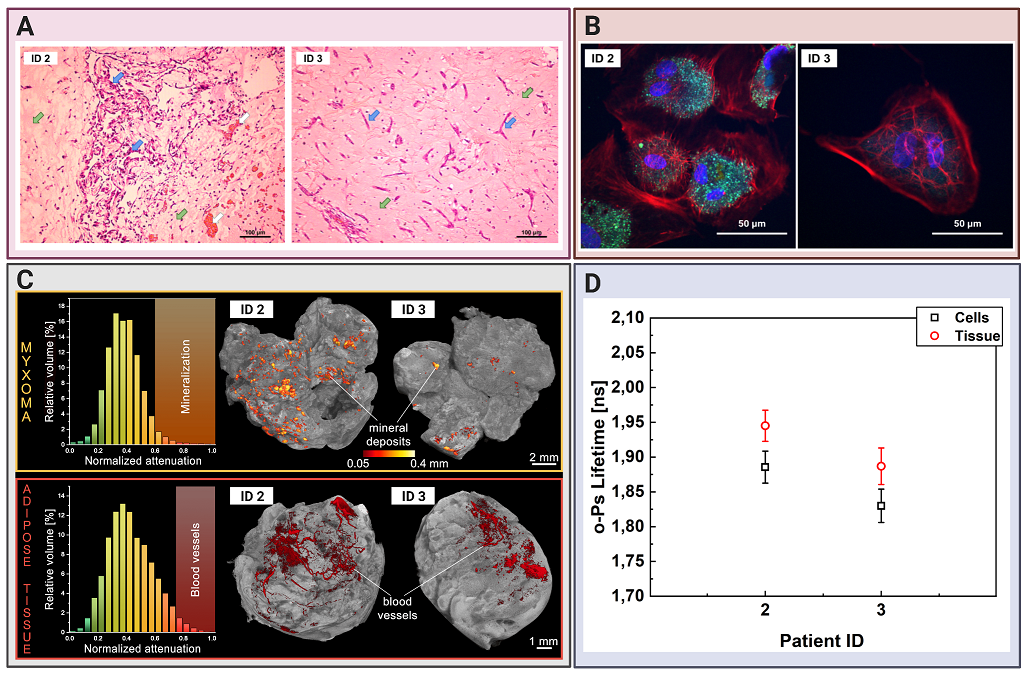
Comparing cardiac myxoma (CM) tissues and the isolated cell line.(A) Micrograph showing exemplary histopathology findings of cardiac myxoma (CM), for patient ID 2 and ID 3 in H&E staining. CM cells stained in purple (blue arrow) can have stellate (ID 3) or globular (ID 2) shape. The red/orange structures (white arrow) correspond to the blood vessels with erythrocytes. The surrounding myxoid matrix is stained in pink (green arrow). (B) Confocal microscopy image with the CM cells stained for F-Actin (red), nucleus (blue), and VE-cadherin (green). The scale bar is 50 μm. (C) Micro-computed tomography results for CM (upper row) and adipose tissues (lower row). Histograms on the left side present normalized X-ray attenuation within the sample: (i) the mineral deposits range from 0.6-1.0 in the CM samples; (ii) the blood vessels range from 0.75-1.0 in the adipose tissue samples. These attenuation ranges have been binarized to extract the mineral deposits and blood vessels for further analysis and visualization. The right side contains volume-rendered 3-D models of the two most representative samples, namely CM and adipose tissues. The internal mineral deposits have been highlighted in the CM model. Its diameter has been color-coded using a heat map. The blood vessels have been colored red in the adipose tissue. (D) Results of the mean ortho-Positronium (o-Ps) lifetime for CM tissue (red circles), isolated from the same patient myxoma cell line (black squares).
More information can be found in:
Bio-medical studies
Positron Annihilation Lifetime Spectroscopy (PALS) is widely used to correlate the mean o-Ps lifetime value with cavity size in which annihilation takes place. PALS makes it possible to study many properties of materials such as the presence of defects, thermal expansion, temperature of phase transitions in polymers, processes of gas, or steams sorption in pores. Unfortunately it was applied in a very limited number of cases concerning living biological material. The PALS method combined with J-PET system will enable the determination of early and advanced stages of carcinogenesis by observing changes in biomechanical parameters between healthy and tumor cells.
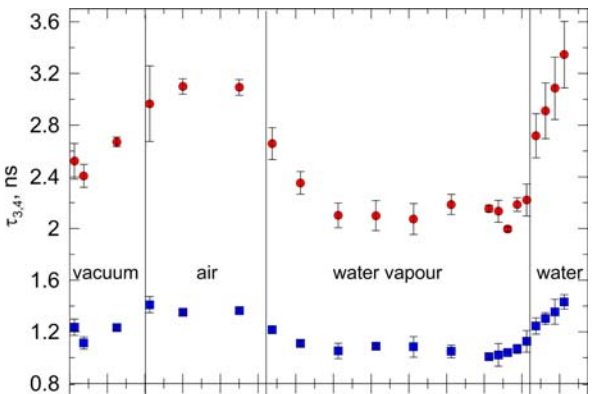
The o-Ps lifetime as a function of the water vapor sorption time. The measurements were conducted in four stages: (1) in vacuum, (2) in dried air, (3) with the presence of water vapor, and (4) with drop of water placed in the chamber containing yeast.
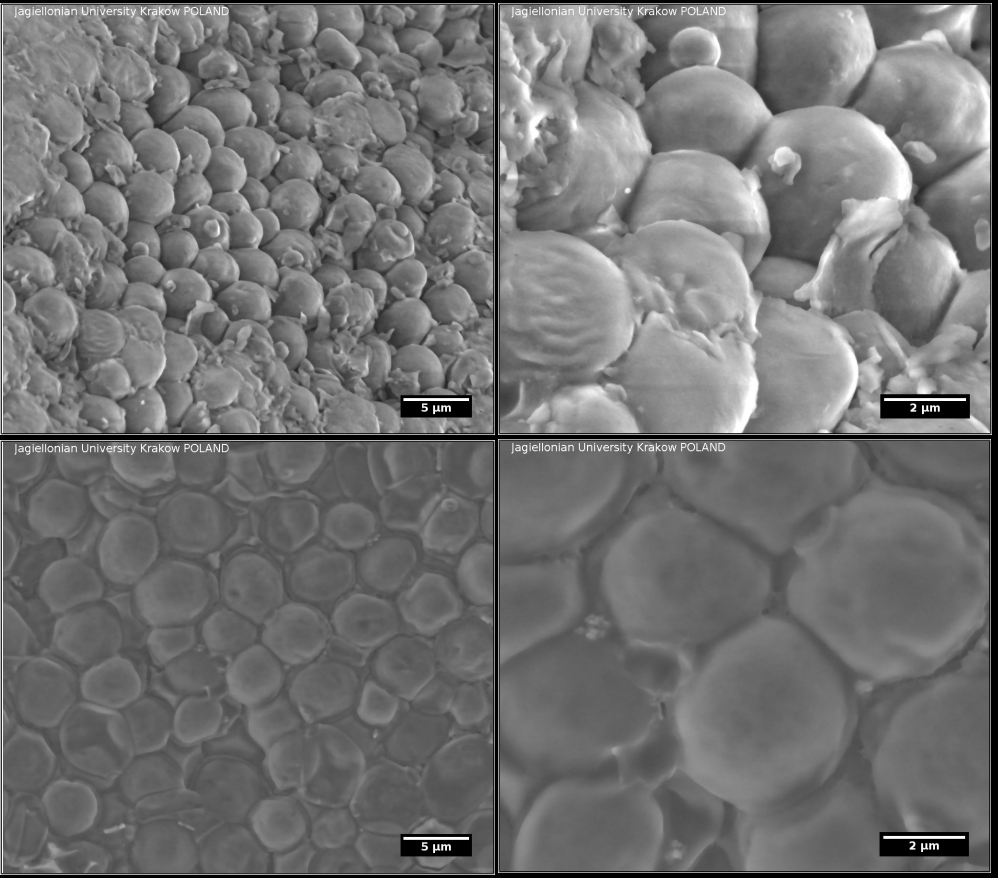
Environmental scanning electron microscopy (ESEM) images of lyophilized yeasts (upper) and dried under normal conditions after addition of water (bottom).
More information can be found in:
- Patent application for a TOF-PET tomograph and a method of imaging using a TOF-PET tomograph, based on a probability of production and lifetime of a positronium,
- Positronium in medicine and biology,
- Studies of unicellular micro-organisms Saccharomyces cerevisiae by means of Positron Annihilation Lifetime Spectroscopy.
Pilot studies with cultured cell lines
Chosen normal (melanocytes) and cancer (melanoma) cell lines were cultured with standard procedures. For the PALS measurement cells were suspended in different freezing mediums, centrifugate and then lyophilised (freeze–dried). The composition of mediums were chosen from standard kryo - preservation reagents (DMSO) and some used in lyophilization process like PROH and trehalose. Viability studies performed before and after freeze-drying, showed best results for cells lyophilised in medium with DMSO and PROH/trehalose.
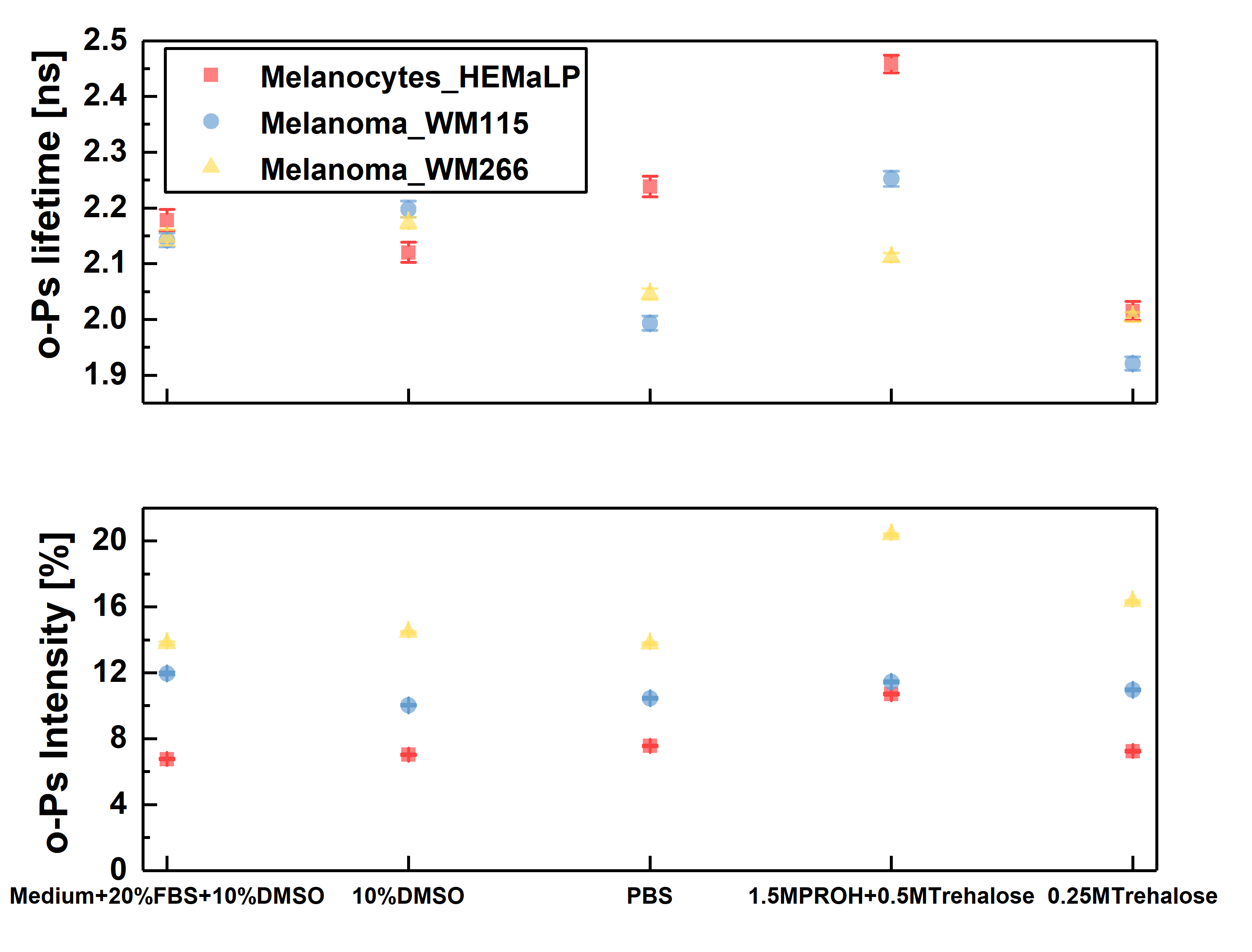
Ortho-positronium lifetime and intensities for cultured cell lines normal – melanocytes (red square), cancer – melanoma WM115 (blue circle), melanoma WM266 (yellow triangle) freeze–dried in different mediums, in order to determine which one will preserve cells the best without changing their structure. The optimal medium was choose to be 1.5 M PROH + 0.5 M Trehalose (based on viability and NMR studies before and after freeze-drying). For this medium significant differences beetwen o-Ps lifetime and intensity can be observed. Cells preserved with DMSO, even though they showed good viability, proved to not be applicable in PALS, since DMSO as a reagent gives strong signal itself.
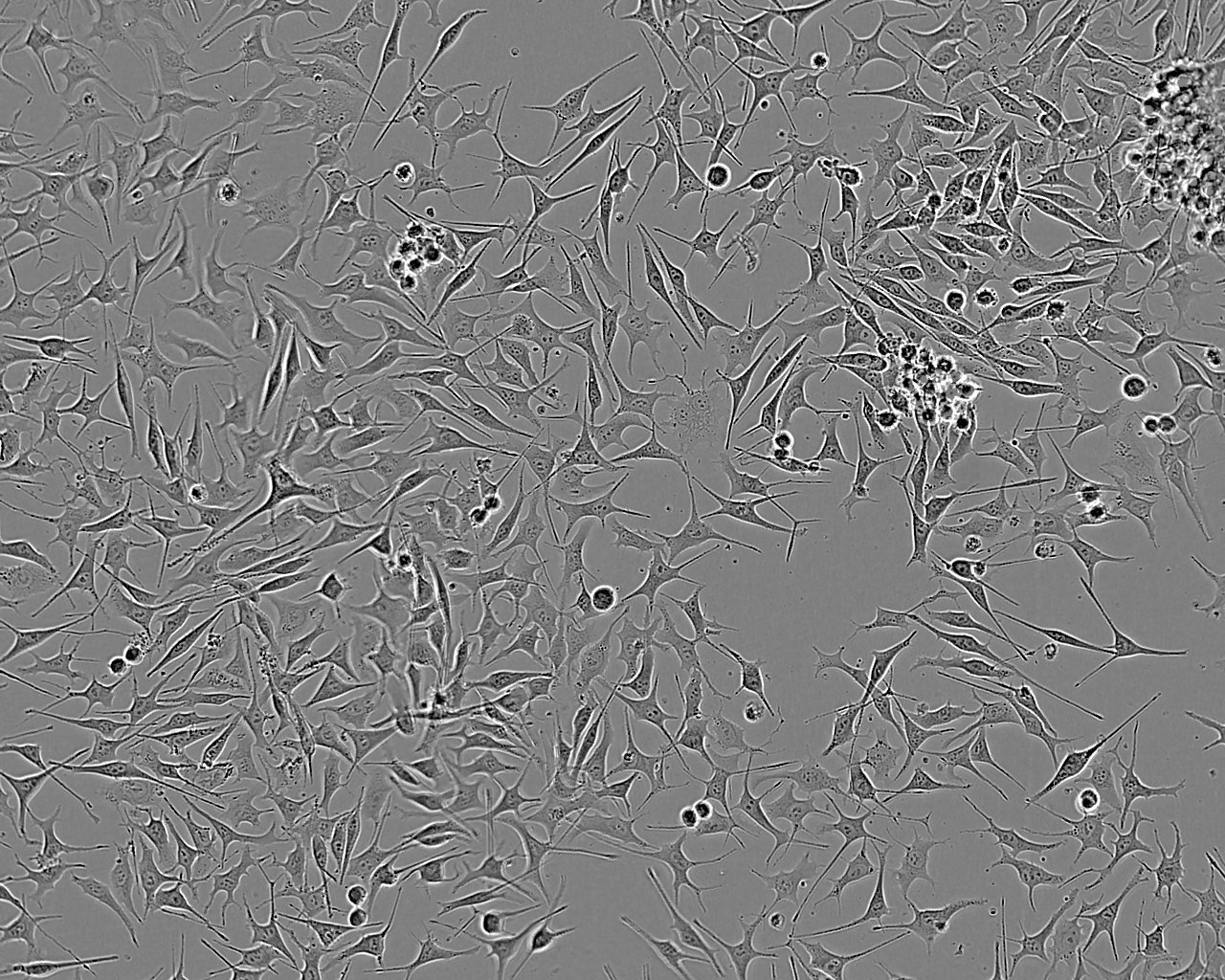
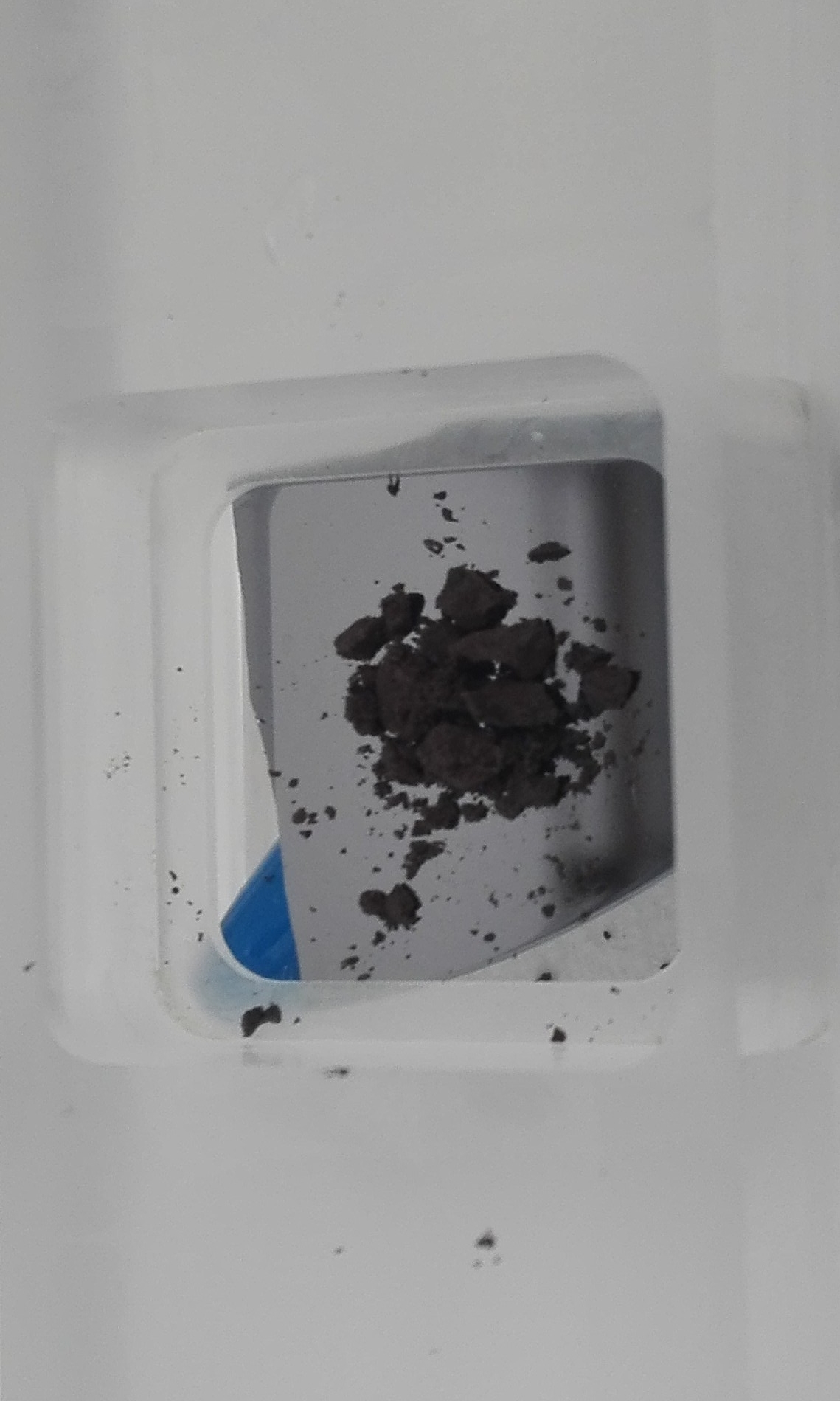
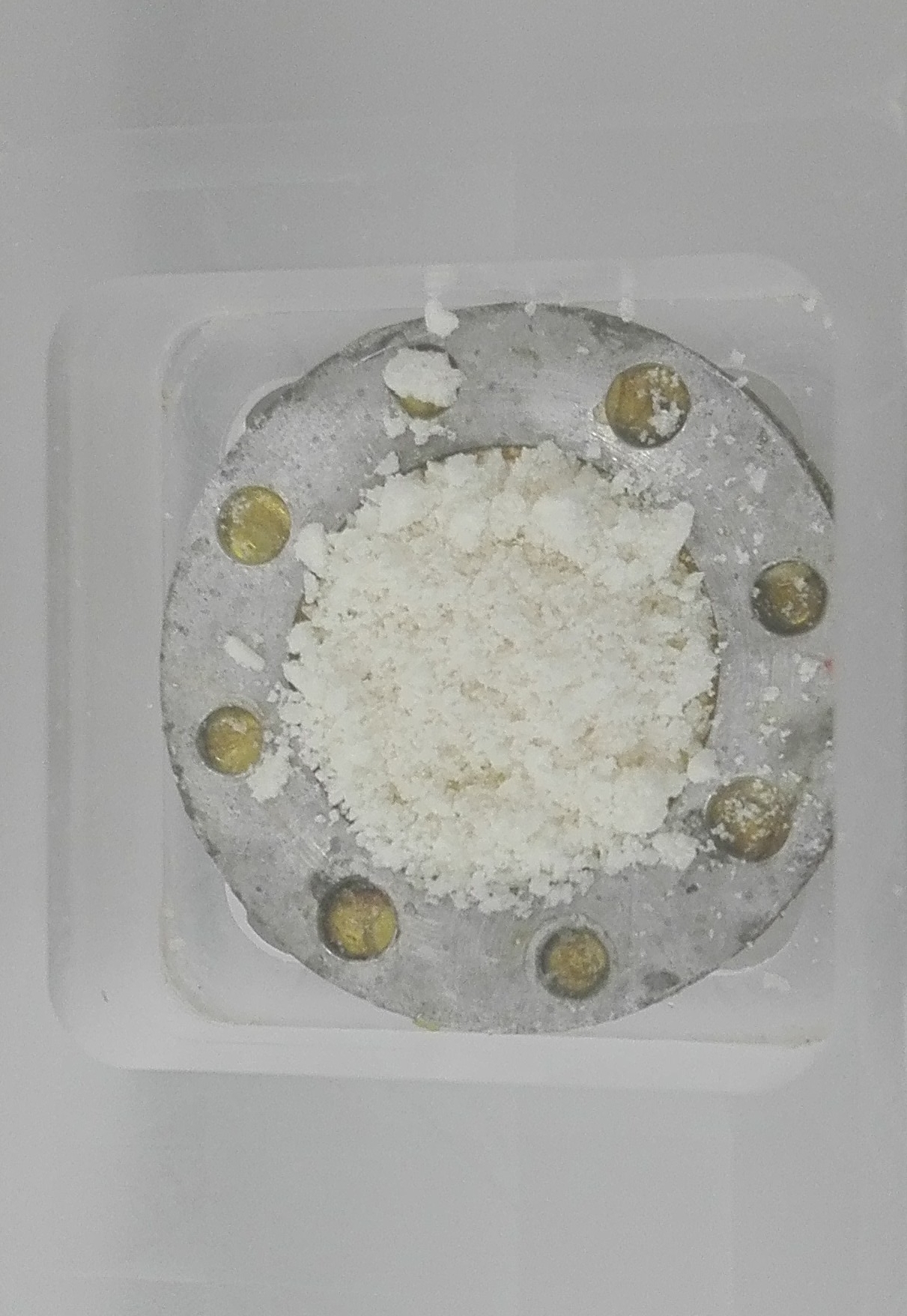
Optical microscope image of melanoma cells in culture (left). Freeze–dried cells prepared for measurement in a holder – melanocytes (middle), melanoma (right).
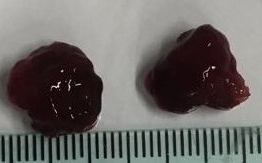
.jpg)
Exemplary photos of a cardiac myxoma tumor (left) and normal adipose tissue (right).
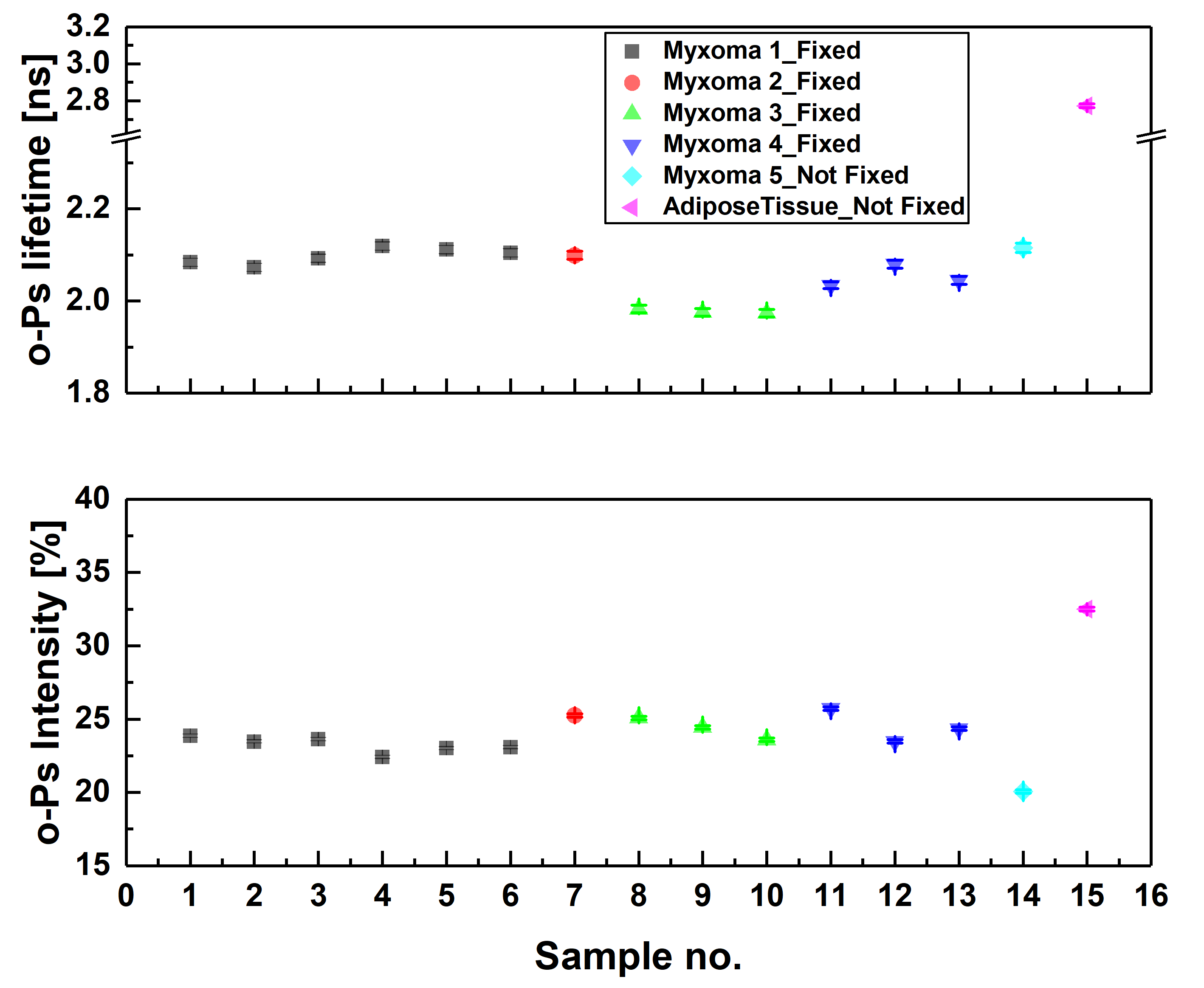
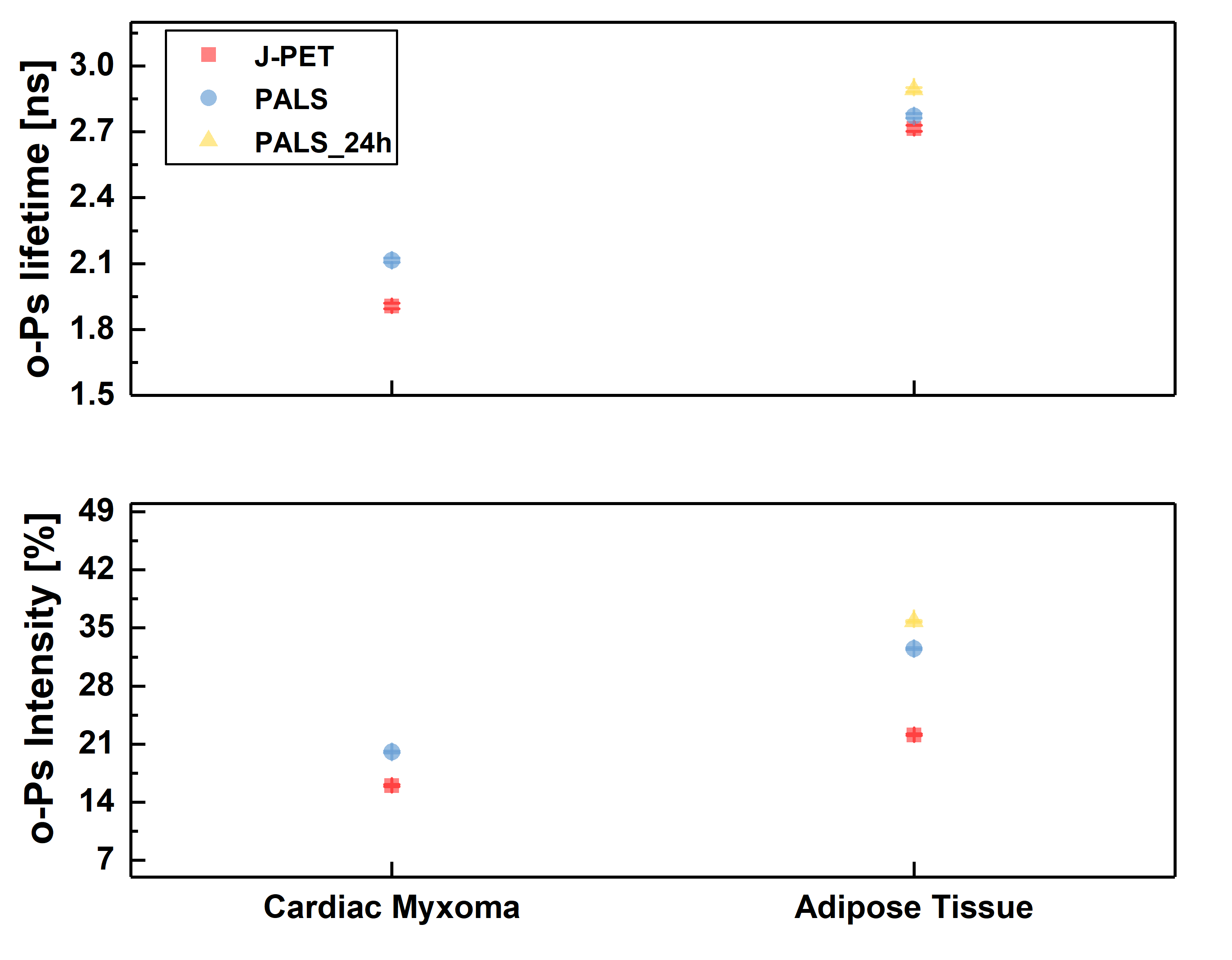
Ortho-positronium lifetime and intensities for (left) cardiac myxoma tumors fixed in formaldehyde/non-fixed and adipose tissue measured on the PALS spectrometer. Results show significance differences between normal and tumor tissue. (right) Non-fixed cardiac myxoma tumor and adipose tissue measured on J-PET and then on PALS. Results for the sample measured on both detectors are consistent with each other, which proves J-PET can be used for morphometric imaging.
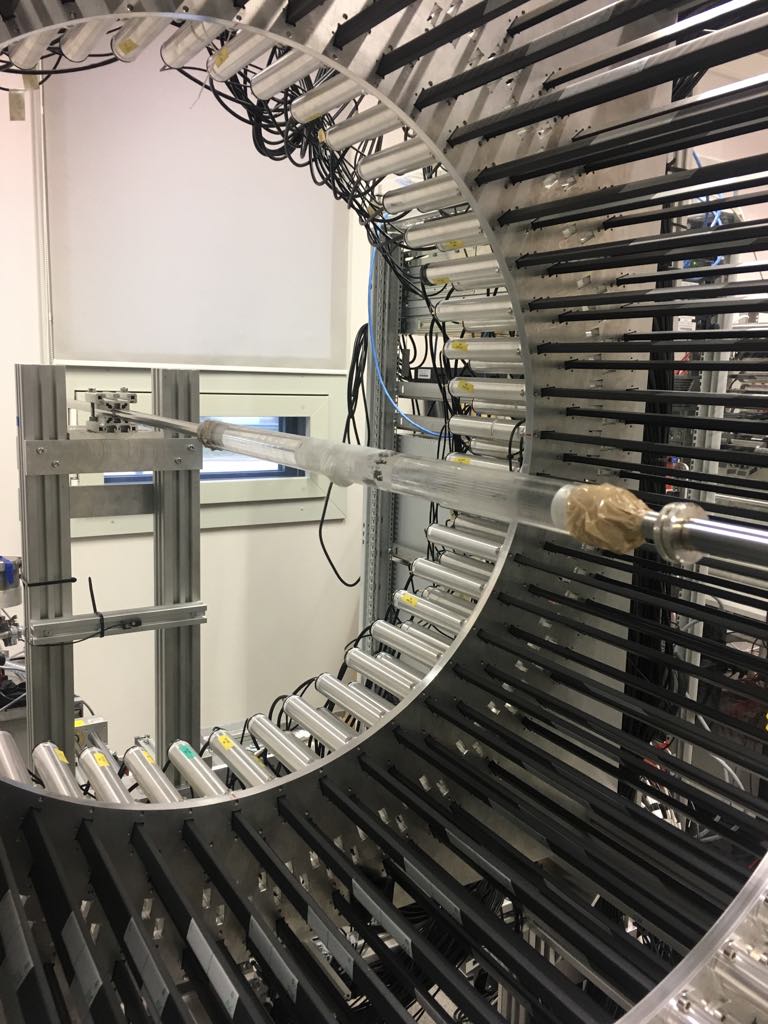
Holder with a sample during the measurement on a standard PALS spectrometer (left) and in J-PET detector (right).
Discreet symmetry studies in positronium decay
Positronium is the lightest purely leptonic object decaying into photons. As an atom bound by a central potential, it is a parity (P) eigenstate and as an atom built out of an electron and an antielectron it is an eigenstate of the charge conjugation (C) operator. Positronium in the ground substates with orbital angular momentum L = 0 is formed in a singlet state of the anti-parallel spins orientation (para-positronium, p-Ps), or in a triplet state of parallel spin orientation (ortho-positronium, o-Ps). Due to the symmetry of charge conjugation p-Ps undergoes annihilation with emission of an even number of photons (most often: two), while o-Ps undergoes annihilation with emission of an odd number of photons (most often: three).
The J-PET detector enables to perform tests of discrete symmetries in the leptonic sector via the determination of the expectation values of the discrete-symmetries odd operators, which may be constructed from the spin of o-Ps and the momenta and polarization vectors of photons originating from its annihilation.
With respect to the previous experiments performed with crystal based detectors, J-PET provides superior time resolution, higher granularity, lower pile-up and an opportunity of determining photon’s polarization. These features allow us to expect improvement by more than an order of magnitude in tests of discrete symmetries in decays of positronium atoms. The CPT symmetry being combination of the charge conjugation C, spatial parity P and reversal in time T, remains poorly tested in charged leptonic sector. The CPT noninvariance effects could be manifested in non-vanishing angular correlationsin the annihilations of the positronium atom - the lightest leptonic bound state. With the Jagiellonian PET (J-PET) prototype, plastic scintillator-based positron-emission-tomography scanner, we were able to measure almost entire available phase-space for ortho-positronium (o-Ps) annihilation into 3 photons. Using a novel technique of single-event estimation of o-Ps spin, the complete spectrum of an angular correlation operator sensitive to CPT-violating effects was determined. We reached the precision of CPT test at the 10−4 level, which is about threefold higher with respect to the previous measurement [NatureComm2021].
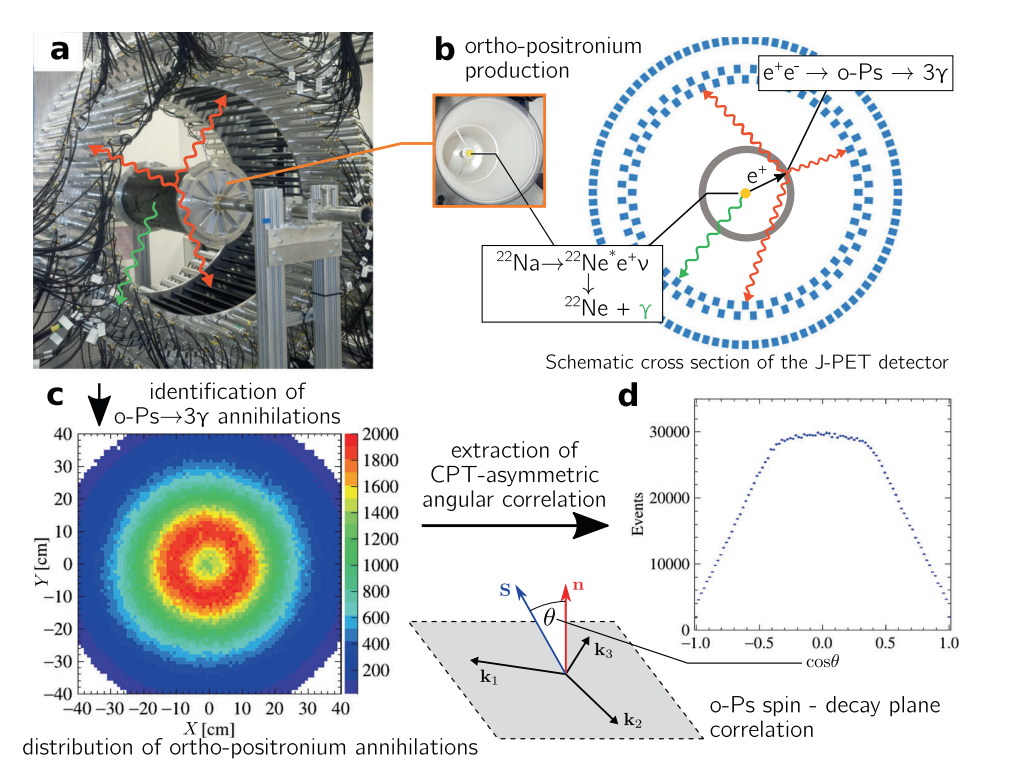
Methodology of the measurement of CPT-violation-sensitive angular correlation in three-photon annihilations of ortho-positronium. a) Photograph of the J-PET tomograph with the cylindrical positronium production chamber. Plastic scintillators (black strips) record photons produced in o-Ps → 3γ events (red arrows) and a prompt photon from β+ emitter de-excitation (green arrow). b) Photograph of the interior of the positronium production chamber and a schematic view of the transverse cross section of the J-PET tomograph. Plastic scintillators (with rectangular cross sections (blue)) form three coaxial rings. Annihilating o-Ps atoms are produced by positrons from a 22Na β+ source in the centre of a cylindrical vacuum chamber (grey), the walls of which are coated with porous silica, where positrons thermalise and form positronia. c) Reconstructed spatial locations of the identified o-Ps →3γevents obtained using three-photon annihilations, allowing us to reproduce a tomographic image of the vacuum chamber. The maximum density distribution is in a ring with a radius of 12 cm, equal to the radius of the positronium production chamber. d) For every selected event, the o-Ps spin axis is reconstructed, allowing calculation of the cosine of the angle between the spin (S) and annihilation plane orientation (n=k1 × k2), the distribution of which is sensitive to CPT-violating asymmetries. The histogram presents the determined cos θ distribution for 1.9 × 106 identified o-Ps → 3γ events.
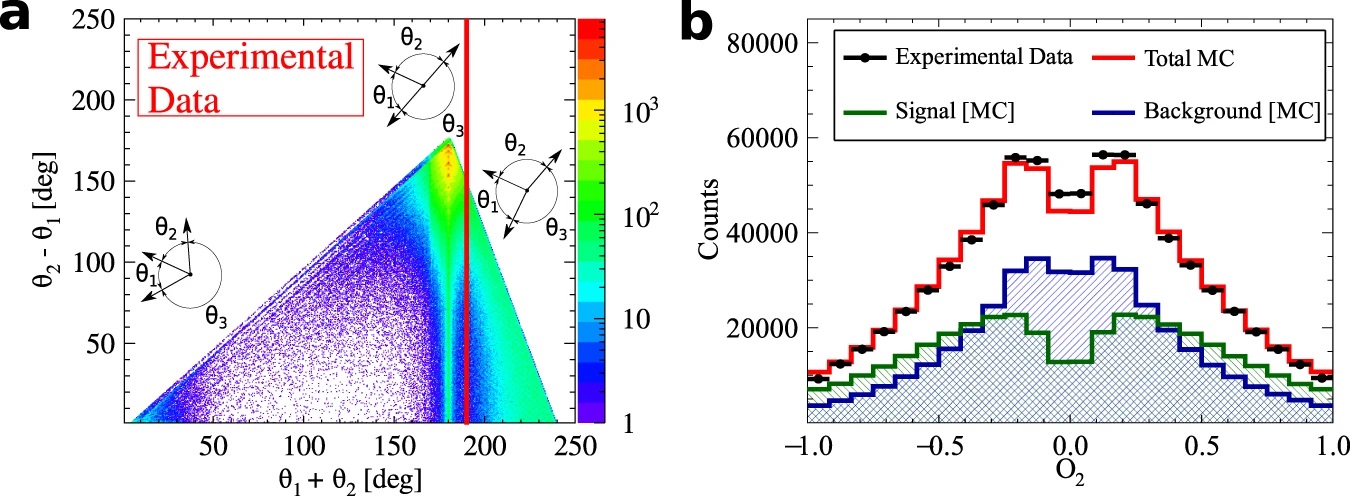
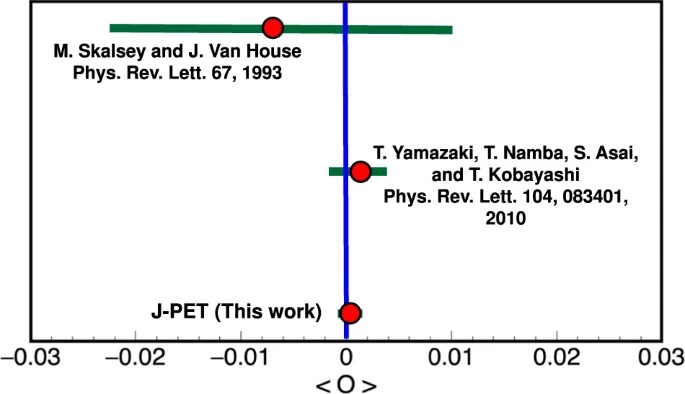
a) Distribution of the sum and difference of the two smallest angles between photon momenta (θ1 < θ2 < θ3). The superimposed black pictographs present three different orientations of the momentum vectors for multiple scattered events (bottom left region), p-Ps→ 2γ events with single scattering (vertical band around θ1 + θ2 = 180°) and o-Ps→ 3γ signal events (bottom right region). The red vertical line corresponds to a θ1 + θ2 ≥ 190° cut applied for the signal selection. b) Measured distribution of asymmetry operator for experimental data (black circles) and simulated histograms for signal (green), background (blue) and combined signal and background (red). The discrepancy between simulated distribution and data points for the two central bins may be explained by the rapid change of efficiency distribution in that region, but this effect is negligible comparing to the achieved accuracy of the final result. Lower panel: Summary of searches for CP-odd ortho-Positronium decays. The two upper results are performed for the operator correlation defined in as O1 = (S⋅k1)(S⋅(k1xk2)), whereas J-PET is using the new correlation constructed with the polarization vector &epsilon i⋅kj. The blue vertical line indicates no CP symmetry violation, while the green bars for each measurement correspond to the total uncertainty calculated as statistical and systematic uncertainties combined in quadrature.
Tests of discrete symmetries with the J-PET tomograph are supported by the National Science Centre through the grants no. 2016/21/B/ST2/01222 and 2019/35/B/ST2/03562.
More information can be found in:
- Discrete symmetries tested at 10−4 precision using linear polarization of photons from positronium annihilations
- Testing CPT symmetry in ortho-positronium decays with positronium annihilation tomography
- Potential of the J-PET Detector for Studies of Discrete Symmetries in Decays of Positronium Atom - A Purely Leptonic System
- popular science description of investigations (wersja po polsku)
Studing the cosmic rays muon puzzle - μPPET
Astroparticle physics is the interdisciplinary field dedicated to understanding how and why the Universe is as it is. Using the theories and models understood and tested in particle physics laboratories, it observes the Universe and the astrophysical sources with different eyes: the particles, also called “the messengers.” The most abundant and easy-to-detect messenger is the gamma rays: almost every source produces them as energy emission; however, they interact too easily and a strong horizon limiting the observations energy appears. The most elusive is the astrophysical neutrinos: whenever there is a weak interaction (i.e., nuclear and particle transformation), they appear; since they are almost unstoppable because of their rare interactions, they come directly from the source. The most pivotal messenger, strongly correlated to the other two, is the Cosmic Rays: nuclei of ejected matter and accelerated to extreme energies (even up to the ZeV!); they keep the information on the source composition, interactions, and its fate, and the Universe composition and chemical element construction (i.e., the formation of all astrophysical objects, including us).
The Cosmic Rays (CRs) interact with all three forces: electromagnetic, weak, and strong. The last one is responsible for their destruction once they reach us and enter in our atmosphere. Their passage produces an “air shower.” As soon as a CR (primary particle) interacts with an air molecule, other particles are generated (“mesons,” mostly Pions and Kaons), which interact as well, creating a particle cascade. The mesons also decay, producing a shower of gammas, electrons, muons (µ), and neutrinos. This process is not eternal because particle daughters subdivide their mother’s energy in each step. Hence, once these secondary particles no longer have enough energy, they become absorbed by the air and disappear. The surviving particles reaching the ground cover an extensive area, even kilometers square! While the density of electrons and gammas reaching the ground delivers the energy information of the primary particle, the muons convey the atomic mass (i.e., what kind of nucleus arrived at us). Both pieces of information are pivotal to understanding the arriving CRs and their diffusion in the Universe. The energy spectrum is well-measured. The mass composition is precisely known only for CRs with energies below 1015 eV –PeV– (HECR), where it is possible to detect the primary directly through satellites. But at higher energies (UHECR), currently up to 1021 eV –ZeV–, they are too rare and energetic for satellites, and we need to rely on indirect measurements, such as the muon density on the ground. The air shower development in the atmosphere is so complicated that we must rely on extensive simulations of the particle-air hadronic interaction to interpret the measurements in terms of mass composition. The current models, however, predict much fewer muons of what we observe in the detectors, making the result interpretation unreasonable. This excess is called the Muon Puzzle. It is considered as such because the early stages of the air shower development have proven correct! Only on the ground muons have an unpredicted excess.
The project µPPET [mu(µ)on Probe with J-PET; pronounced as the word /muppet/] plans to investigate this puzzle by studying the trajectories of the muons –and other observables, such as their charge distribution–. That is the first experiment dedicated solely to studying it. All Cosmic Ray experiments have tried to solve it so far. Still, there are too many unknown variables (mass composition), and the detector’s geometry is optimized for different scientific cases. In particular, the polarization effects from the geomagnetic field is considered in the hadronic interaction models. The polarization of the projectile (i.e., a muon) and/or the target (air molecule) introduces a small correction to the probability of muon interaction, i.e., their production and their trajectories. In the early stages of the air shower, it is negligible, passing unnoticed experimentally. However, having this correction cumulated interaction after interaction, the number of muons is expected to be larger on the ground. µPPET will make full use of both the J-PET: the Big Barrel will be used as a precise muon tracker, while the modules of the Modular one will be scattered on the campus roofs to form an array to reconstruct the air shower geometry (see Figure as a preliminary geometry). The project will follow two parallel lines of work: the modeling of the hadronic models and the measurements.
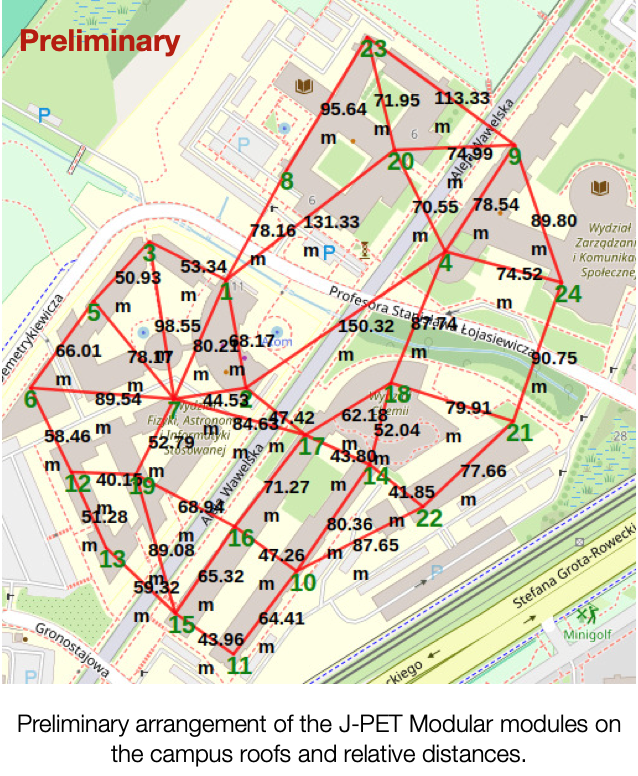
The project µPPET is supported by the National Science Centre through the grant no. 2023/50/E/ST9/00576.
More information can be found in:
Materials science
Together with research and development of the J-PET scanners new scintillating materials with properties suitable for time-of-flight PET devices are synthesized and investigated [BioAlgMedSys2014]. The aim of the research is to develop plastic scintillators with shorter decay time [PLOSOne2017] and higher light output [ActaPhysPolB2020] properties than those commercially available.
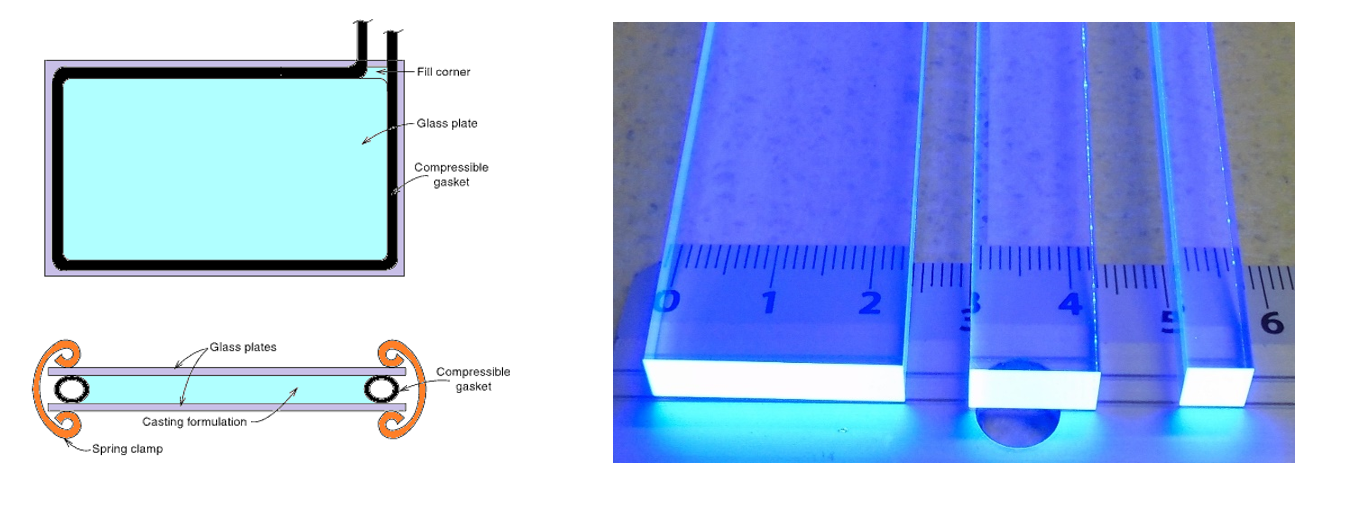
Left: Face and edge views of conventional cell casting mold configuration for plastic scintillator manufacturing. Right: Three EJ-200 plastic scintillators with different cross-sections excited under a UV lamp.
Plastic scintillator is an optical material converting ionizing radiation into light. It consist of two fluorescent dyes dissolved in polymeric base [BioAlgMedSys2021]. Polymer-based scintillators emit about 10 000 blue photons per MeV of absorbed radiation energy with short pulse duration in range of few nanoseconds [RadiatMeas2022]. Fast timing of signals are foundation of time-of-flight PET scanners, for example J-PET scanner based on fast plastic scintillators.
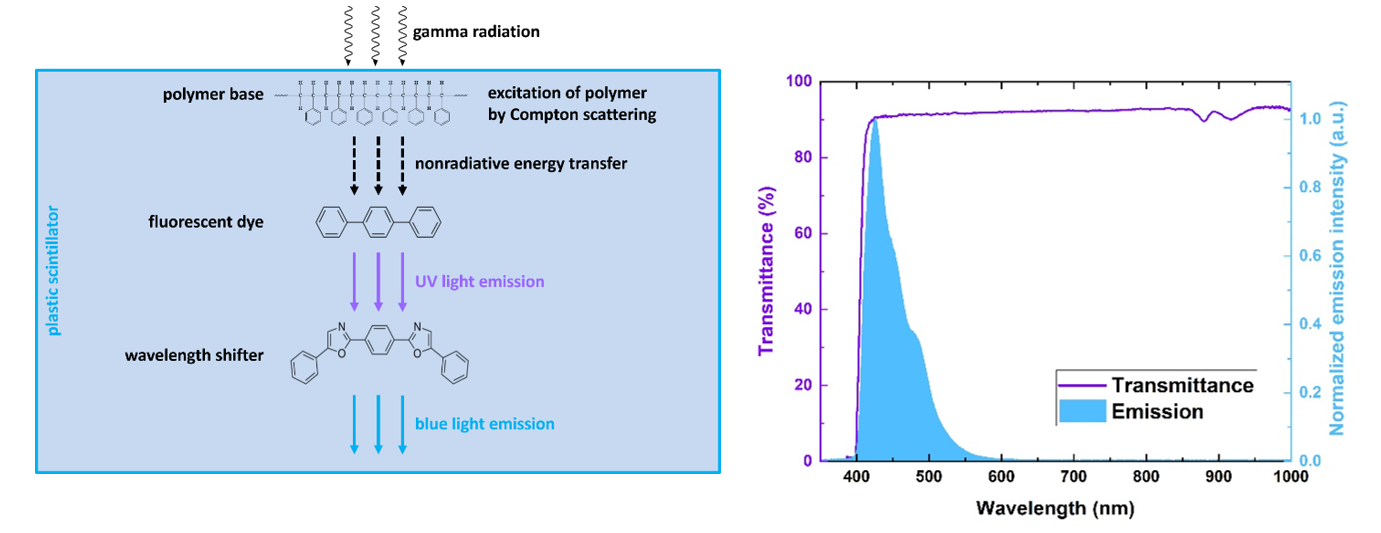
Left: Energy flow in plastic scintillators excited by gamma radiation: energy from gamma quanta is converted into blue light in three steps via fluorescence mechanism. Right: Example of transmission spectrum of BC-408 plastic scintillator strip superimposed on its emission spectrum.
Additionally, large transparency of plastic scintillator material allows the construction of time-of-flight J-PET scanners with long field-of-view [IEEE2020]. High transparency of the plastic scintillators increases also time resolution of the total-body J-PET scanner because more light reaches silicon photomultipliers attached at both ends of the plastic scintillator strip [NIMA2023].
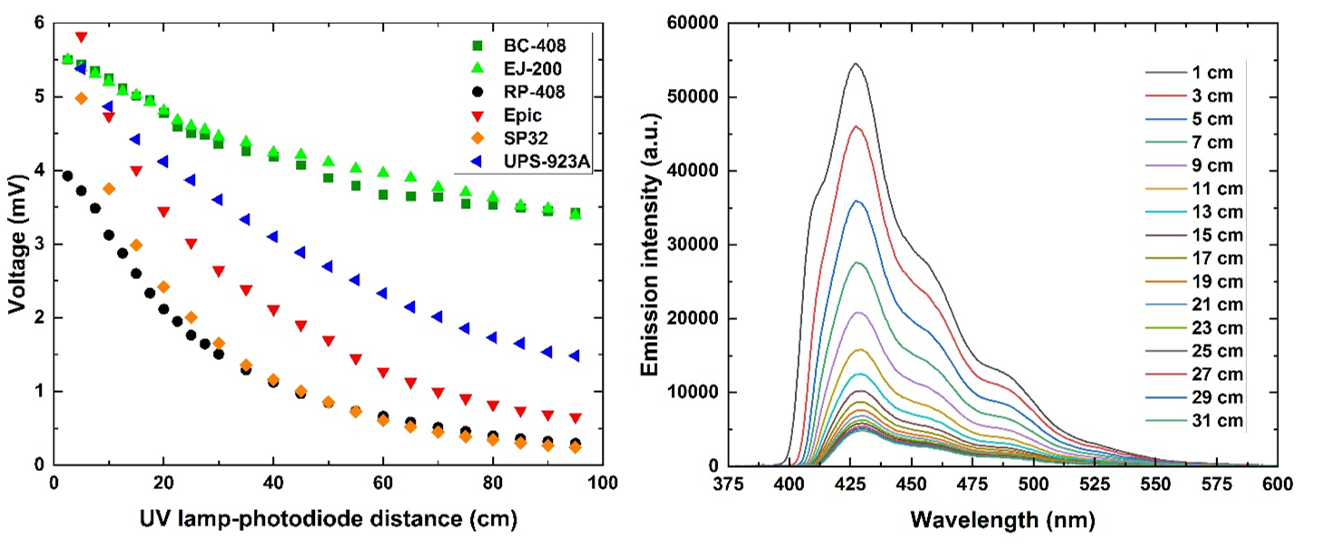
Left: Comparison of technical attenuation of light signals measured for various plastic scintillator types. Right: Emission spectra of BC-408 plastic scintillator change with excitation source-scintillator end edge distance.
More information can be found in:
List of articles
Concept The first positronium imaging of human tissues ex-vivo and in-vivo-
Positronium image of the human brain in vivo
Science Advances 10 (2024) eadp2840 -
Positronium imaging with the novel multiphoton PET scanner
Science Advances 7 (2021) eabh4394
- Discrete symmetries tested at 10−4 precision using linear polarization of photons from positronium annihilations
Nature Communications 15 (2024) 78 -
Testing CPT symmetry in ortho-positronium decays with positronium annihilation tomography
Nature Communications 12 (2021) 5658
-
Colloquium: Positronium physics and biomedical applications
Rev. Mod. Phys. 95 (2023) 021002 -
Positronium in medicine and biology
Nature Reviews Physics 1 (2019) 527
-
Nonmaximal entanglement of photons from positron-electron annihilation demonstrated using a novel plastic PET scanner
Science Advances 11 (2025) eads3046 -
Witnessing Entanglement In Compton Scattering Processes Via Mutually Unbiased Bases
Nature: Scientific Reports 7 (2017) 15349 -
Feasibility studies of the polarization of photons beyond the optical wavelength regime with the J-PET detector
Eur. Phys. J. C 78 (2018) 970
- Feasibility of the J-PET to monitor the range of therapeutic proton beams
Physica Medica 118 (2024) 103301 - Detection of range shifts in proton beam therapy using the J-PET scanner: a patient simulation study
Phys. Med. Biol. 68 (2023) 145016 -
Experience and new prospects of PET imaging for ion beam therapy monitoring
Z. Med. Phys. 33 (2023) 22 - J-PET application as a Compton camera for proton beam range verification: A preliminary study Bio-Algorithms and Med-Systems 19 (2023) 23
-
ProTheRaMon - a GATE simulation framework for proton therapy range monitoring using PET imaging,
Phys. Med. Biol. 67 (2022) 224002
-
Developing a Novel Positronium Biomarker for Cardiac Myxoma Imaging
EJNMMI Phys. 10 (2023) 22 -
3D melanoma spheroid model for the development of positronium biomarker
Sci. Rep. 13 (2023) 7648 -
Perspectives on translation of positronium imaging into clinics
Front. Phys. 10 (2022) 969806 -
Positronium as a biomarker of hypoxia
Bio-Algorithms and Med-Systems 17(4) (2021) 311
-
Simulating NEMA characteristics of the modular total-body J-PET scanner - an economic total-body PET from plastic scintillators
Phys. Med. Biol. 66 (2021) 175015 -
Estimation of the NEMA characteristics of the J-PET tomograph using the GATE package
Phys. Med. Biol. 63 (2018) 165008
- The J-PET detector-a tool for precision studies of ortho-positronium decays
Nuclear Inst. and Methods in Physics Research A 1008 (2021) 165452 - Feasibility study of the positronium imaging with the J-PET tomograph
Phys. Med. Biol. 64 (2019) 055017 - Concept: patent application TOF-PET tomograph and a method of imaging using a TOF-PET tomograph, based on a probability of production and lifetime of a positronium
-
Trilateration-based reconstruction of ortho-positronium decays into three photons with the J-PET detector
Nuclear Inst. and Methods in Physics Research A 819 (2016) 54 - A feasibility study of ortho-positronium decays measurement with the J-PET scanner based on plastic scintillators
Eur. Phys. J. C 76 (2016) 445 -
Determination of the 3gamma Fraction from Positron Annihilation in Mesoporous Materials for Symmetry Violation Experiment with J-PET Scanner
Acta Phys. Polon. B 47 (2016) 453
-
Evaluation of Single-Chip, Real-Time Tomographic Data Processing on FPGA - SoC Devices
IEEE Trans. Med. Imaging. 37 (2018) 2526 -
Multichannel FPGA based MVT system for high precision time (20 ps RMS) and charge measurement
JINST 12 (2017) P08001 -
Sampling FEE and Trigger-less DAQ for the J-PET Scanner
Acta Phys. Polon. B 47 (2016) 491 (2016) -
Overview of the software architecture and data flow for the J-PET tomography device
Acta Phys. Polon. B 47 (2016) 561
-
Measurement of gamma quantum interaction point in plastic scintillator with WLS strips
Nuclear Instruments and Methods in Physics Research A 851 (2017) 39
-
Time resolution of the plastic scintillator strips with matrix photomultiplier readout for J-PET tomograph
Phys. Med. Biol. 61 (2016) 2025
-
Scatter Fraction of the J-PET Tomography Scanner
Acta Phys. Polon. B 47 (2016) 549 -
Multiple scattering and accidental coincidences in the J-PET detector simulated using GATE package
Acta Phys. Pol A 127 (2015) 1505
-
Calculation of the time resolution of the J-PET tomograph using kernel density estimation
Phys. Med. Biol. 62 (2017) 5076 -
A novel method for the line-of-response and time-of-flight reconstruction in TOF-PET detectors based on a library of synchronized model signals
Nuclear Inst. and Methods in Physics Research A 775 (2015) 54 -
Hit time and hit position reconstruction in the J-PET detector based on a library of averaged model signals
Acta Phys. Pol A 127 (2015) 1495
-
Potential of the J-PET Detector for Studies of Discrete Symmetries in Decays of Positronium Atom - A Purely Leptonic System
Acta Phys. Polon. B 47 (2016) 509
-
A novel method for the line-of-response and time-of-flight reconstruction in TOF-PET detectors based on a library of synchronized model signals
Nuclear Inst. and Methods in Physics Research A 775 (2015) 54 -
Test of a single module of the J-PET scanner based on plastic scintillators
Nucl. Instr. and Meth. A 764 (2014) 317
- Comparative studies of plastic scintillator strips with high technical attenuation length for the total-body J-PET scanner
Nucl. Instrum. Meth. A 1051 (2023) 168186 - Investigation of the light output of 3D-printed plastic scintillators for dosimetry applications
Radiat. Meas. 158 (2022) 106864 - Blue-emitting polystyrene scintillators for plastic scintillation dosimetry
Bio-Algorithms and Med-Systems 17 (2021) 191 - Synthesis and characterization of plastic scintillators for the total-body J-PET scanner
Acta Phys. Polon. B 51 (2020) 225 - Technical attenuation length measurement of plastic scintillator strips for the total-body J-PET scanner
IEEE Trans. Nucl. Sci. 67 (2020) 2286 - Novel scintillating material 2-(4-styrylphenyl)benzoxazole for the fully digital and MRI compatible J-PET tomograph based on plastic scintillators
PLOS ONE 12 (2017) e0186728 - PALS investigations of free volumes thermal expansion of J-PET plastic scintillator synthesized in polystyrene matrix
NUKLEONIKA 60 (2015) 777 - Plastic scintillators for positron emission tomography obtained by the bulk polymerization method
Bio-Algorithms and Med-Systems 10 (2014) 27
Members
Former members
Recent publications
- muPPET: Investigating the Muon Puzzle with J-PET Detectors
- In Vivo Intralesional Positronium Lifetime Imaging of Thyroid Cancer Using Clinically Routine I-124 PET/CT
- Positronium Imaging: History, Current Status, and Future Perspectives
Recent theses
- Testing CPT symmetry in ortho-positronium decays at the precision of 10^-4 with J-PET
- Studies of quantum entanglement in pair of high energetic photons from e+e? annihilation
- Determining positronium lifetimes in uniform samples of aluminum, copper, nickel and quartz using modular J-PET scanner and Biograph Vision Quadra scanner
- Evaluation of the NEMA characteristics for the Modular J-PET scanner